Chapter 23 Blood and bone marrow
COMMON CLINICAL PROBLEMS FROM BLOOD AND BONE MARROW DISEASE
Pathological basis of haematological signs and symptoms
Sign or symptom | Pathological basis |
---|---|
Tiredness, dyspnoea | Reduced oxygen-carrying capacity of blood due to anaemia |
Mucosal pallor | Anaemia |
Glossitis (sore mouth, smooth tongue) | Mucosal effects of haematinic deficiency |
Spoon-shaped nails | Due to iron deficiency |
Jaundice | Bilirubin accumulation from haemolysis |
Abnormal tendencyto infections | Neutropenia, e.g. in leukaemia or hypoplastic anaemiaImmune deficiency, e.g. in myeloma, and due to chemotherapy in leukaemia and lymphoma |
Splenomegaly | Due to expansion of haemopoiesis in myeloproliferative disorders, red cell pooling and destruction in haemolytic anaemias, infiltration in leukaemias and lymphomas |
Also non-haematological causes, e.g. portal hypertension | |
Lymphadenopathy | Non-neoplastic causes, e.g. infectious mononucleosisInfiltration with leukaemia or lymphoma |
Bone pain and fractures | Osteoclast activation in myeloma |
Purpura, bruising,mucosal or traumaticbleeding | Thrombocytopenia or platelet dysfunction |
Bruising, muscle and joint bleeding, andtraumatic bleeding | Coagulation factor deficiency |
CELLULAR COMPONENTS
The peripheral blood is investigated by microscopy of a droplet spread evenly over the surface of a glass slide—the blood film. Routinely, the blood film is treated with a combination of stains which allow identification of nuclear and cytoplasmic detail (Fig. 23.1).
Erythrocytes
Erythrocytes (red blood cells) are deformable, non-nucleated and biconcave discs (Fig. 23.2). They are the most abundant blood cell. When blood is separated, by centrifugation, into cellular and plasma components, the red cell portion is approximately 45% of the total volume: this is the ‘packed cell volume’ or haematocrit.
The erythrocyte is a special oxygen-carrying cell because it is rich in haemoglobin. The cell membrane is composed of a phospholipid bilayer with integral proteins. The shape of the cell is maintained by structural proteins, such as spectrin, which form a cytoskeleton. Enzyme systems protect the haemoglobin from irreversible oxidation. The mature erythrocyte has no nuclear material, so new protein cannot be synthesised. The mature erythrocyte circulates for around 120 days before it is removed by the reticulo-endothelial system.
Absolute values
In the modern laboratory, automated cell counters provide these data on each blood sample analysed.
Changes in disease
Anaemia is present when the haemoglobin concentration is less than approximately 130g/l in a male or 115g/l in a female (Table 23.1); the haematocrit is also reduced. Conversely, polycythaemia describes an increased red cell concentration; it is usually accompanied by a raised haemoglobin concentration and haematocrit.
Anaemias may be simply classified according to red cell size (MCV) and haemoglobin content (MCH). This classification is of great diagnostic value in most common types of anaemia (Table 23.2). Further diagnostic information is obtained by the microscopic examination of the red cell morphology on a blood smear. Disease of the blood is frequently associated with increased variation in red cell size—anisocytosis—and the presence of erythrocytes of abnormal shape—poikilocytosis (Fig. 23.3). Increased erythrocyte anisocytosis and poikilocytosis are non-specific abnormalities present in many haematological and systemic disorders. An example is the marked aniso-poikilocytosis that occurs in the absence of a functioning spleen, due to surgical removal or secondary to disease. In this situation there are also inclusions in red cells. They are called Howell–Jolly bodies (Fig. 23.4) and are remnants of nuclear material that would normally be removed when newly formed erythrocytes released from bone marrow circulate for the first time through the spleen.
In addition to Howell–Jolly bodies, red cells may contain other inclusions (Fig. 23.4) under certain circumstances. The basophilic stippling of the ‘stipple cell’ is due to the presence of residual RNA; stipple cells may be present in several anaemias, especially thalassaemia. Siderotic granules contain iron and may occur in states of iron overload, for example in chronically anaemic subjects who have received treatment by frequent transfusion of red cells. Occasionally, nucleated red cell precursors may escape into the peripheral blood; when these normoblasts are accompanied by immature neutrophil leukocytes the film is described as leukoerythroblastic. A leukoerythroblastic blood film results from gross marrow disturbance such as infiltration by malignancy, or fibrous tissue (myelofibrosis) or in severe anaemia due to deficiency of vitamin B12 or folate (megaloblastic anaemia).
Leukocytes
The normal concentrations of these are:
Neutrophil granulocytes
Neutrophils are the most numerous leukocytes in the blood of the healthy adult. The nucleus of the neutrophil granulocyte is characteristically segmented into up to five lobes and the nuclear chromatin stains densely (Fig. 23.1). The abundant cytoplasm stains pink and contains characteristic granules. Within the granules are enzymes, including myeloperoxidase, alkaline phosphatase and lysozyme. Neutrophils have a scavenging function and are of particular importance in defence against bacterial infection.
Neutrophil precursors and neutrophils spend 14 days in the bone marrow, whereas the half-life of neutrophils in the blood is only 6–9 hours. Peripheral blood counts therefore measure less than 10% of the total body neutrophils. Within the circulation the cells move between a circulating and a ‘marginating’ pool, margination being attachment to vascular endothelial cells. To perform their scavenging function, granulocytes irreversibly enter the tissues by penetrating endothelial cells modified by inflammatory mediators. Cytokine-stimulated endothelial cells present adhesion molecules which interact with neutrophils and facilitate their passage: one such is ICAM-1 (intercellular adhesion molecule 1).
Lymphocytes
The peripheral blood lymphocytes are small leukocytes with a round or only slightly indented nucleus and scanty sky-blue-staining cytoplasm which may contain an occasional pink- or red-staining granule. Circulating B- and T-cells are not distinguishable by morphology alone. Immunological staining shows that in health approximately 70% of circulating small lymphocytes are T-cells and 30% B-cells.
A small proportion of lymphocytes may be larger with abundant cytoplasm, sometimes referred to as ‘activated’ lymphocytes. These are believed to represent cells that have been stimulated, perhaps by foreign antigen. A more complete description of the classification and role of lymphocytes is to be found in Chapter 9.
Changes in disease
Quantitative changes
Causes of reactive neutrophil leukocytosis include:
Monocytosis may be reactive to:
Eosinophil leukocytosis may be reactive to:
The atypical cells in peripheral blood are recognisable as lymphocytes but are much larger and have abundant cytoplasm and nuclear irregularities (Fig. 23.5). They are probably reactive T-lymphocytes responding to B-lymphocytes containing the virus, are detectable in blood about 7 days after the onset of illness and may persist for 6 weeks or more. Apparently fortuitously, but usefully, antibodies reactive against horse, sheep and ox red cells (heterophile antibodies) typically develop during the second week and may persist for a few months; they are detected in the Paul–Bunnell test or by more convenient commercial screening slide tests such as the ‘Monospot’ test, and are of diagnostic value. A very similar clinical and haematological (but not serological) picture can develop as a result of other infections, especially with human immunodeficiency virus (HIV), cytomegalovirus and toxoplasma.
All of the above are examples of reactive leukocytosis. Increased white cell counts in peripheral blood, often with immature forms present, are also a typical feature of some primary disorders of the bone marrow, especially leukaemias and myeloproliferative disorders.
Important causes of selective neutropenia are:
Neutropenia with counts of less than 0.5 × 109/l may result in severe sepsis, especially of the mouth, pharynx (Fig. 23.6) and peri-anal regions, and also in disseminated infection. This clinical picture is now most commonly seen in patients receiving drug or irradiation therapy for malignant disorders.
Qualitative changes
Qualitative leukocyte changes are less important than quantitative abnormalities. Defects of phagocytic cell function resulting in an increased tendency to bacterial infection are recognised, particularly as acquired defects after splenectomy, in leukaemic disorders and due to corticosteroid therapy. Congenital abnormalities of leukocyte function are uncommon. ‘Atypical’ lymphocytes in infectious mononucleosis have been described earlier. Other abnormalities of neutrophil morphology are also recognised (Fig. 23.7).
Platelets
On a stained blood film platelets appear as non-nucleated fragments of granular cytoplasm, approximately one-fifth the diameter of erythrocytes and in a concentration of 150–400 × 109/l. Platelets are contractile and adhesive cells, the function of which is the maintenance of vascular integrity. Exposure of vascular subendothelial structures results in rapid adhesion of platelets to the exposed area and aggregation of platelets to each other in the formation of a primary haemostatic plug (Fig. 23.8). Platelets are rich in intracellular granules, which are released during stimulation. The most abundant granules, alpha granules, contain proteins and peptides, including von Willebrand factor, some coagulation factors and growth factors. Platelets deliver these to sites of vascular injury, where they contribute to clot formation and the repair process. Dense bodies are less abundant platelet granules and are rich in calcium, serotonin and adenine nucleotides.
Thrombocytosis may also occur in primary disorders of bone marrow—the myeloproliferative diseases and chronic myeloid leukaemia. Morphological platelet abnormalities are of minor importance, although ‘giant’ platelets, with a diameter exceeding that of an erythrocyte, are a feature of the myeloproliferative disorders rather than reactive thrombocytosis. Giant platelets are also a feature of some inherited syndromes, including those associated with mutations of the myosin heavy chain gene.
BLOOD PLASMA
Blood coagulation
For normal homeostasis, blood must be fluid; however, the capacity to minimise loss of blood through breaches of the vascular system is essential. The rapid plugging of defects in small vessels is the function of platelets (primary haemostasis, Fig. 23.8) but a more permanent and secure seal results from the generation of insoluble fibrillar fibrin from its soluble plasma protein precursor fibrinogen in the process of blood coagulation. Failure of primary haemostasis, due to platelet disorders, or of coagulation due to clotting factor deficiency or presence of a coagulation inhibitor, can each result in life-threatening haemorrhage. In contrast, inappropriate activation of platelets or blood coagulation may result in vascular occlusion, ischaemia and tissue death. A complex system of activators and inhibitors in plasma has therefore evolved in order to allow localised clot formation at sites of injury but to minimise the risk of inappropriate and undesirable clotting, i.e. thrombosis. These are the coagulation and fibrinolytic factors and their inhibitors (Fig. 23.9).
Important features of the haemostatic mechanism are as follows.
Although the scheme for the initial stages of coagulation activation can be conveniently divided into extrinsic and intrinsic pathways (Fig. 23.9), this is a simplification. Coagulation activation in vivo is initiated through tissue factor, an integral cell membrane protein which is not expressed by vascular endothelial cells in an unstimulated state but is expressed by subendothelial cells and smooth muscle as well as other cells. As soon as blood leaks from a vessel it is exposed to tissue factor. Tissue factor activates factor VII. The much slower pathway for fibrin generation through activation of factor XII on contact with subendothelial components is of minor importance. This partially explains the absence of any increased tendency to haemorrhage in subjects who are congenitally deficient in factor XII. It is the tissue factor–activated factor VII complex that rapidly activates factors X and IX, leading to thrombin generation. When the procoagulant stimulus is sufficiently strong, the degree of amplification through thrombin activation of factors V and VIII overcomes inhibition by activated protein C and fibrin generation proceeds.
HAEMOPOIESIS AND BLOOD CELL KINETICS
Haemopoiesis is the formation of blood cells.
Sites of haemopoiesis
In the adult, all blood cells are produced in the red marrow, which is restricted to the bones of the axial skeleton—vertebrae, ribs, sternum, skull, sacrum, pelvis and proximal femora. In these regions the bone marrow is composed of approximately 50% fat, within adipocytes, and 50% blood cells and their precursors (Fig. 23.10). The fatty marrow of other bones is capable of haemopoiesis when requirements for blood cells are increased in some diseases.
In the infant and young child, practically all of the bones contain haemopoietically active marrow.
The bone marrow is examined histologically in two ways. Marrow can be aspirated through a needle inserted into a marrow cavity (usually sternum or pelvis), smeared on a slide and stained in a method similar to that for peripheral blood. Further information, particularly on the structure and cellularity of the marrow, can be obtained by preparation of sections of a marrow trephine biopsy: this is a core of tissue obtained using a wide-bore needle (Fig. 23.10).
Haemopoietic stem cells
Studies of bone marrow in culture lead to the conclusion that erythrocytes, leukocytes (including lymphocytes) and platelets are derived from a common, self-replicating precursor cell or ‘pluripotential stem cell’. By a series of cell divisions, cells committed to each line are produced and further divisions result in mature cells—erythrocytes, granular leukocytes, megakaryocytes and T- and B-lymphocytes (Fig. 23.11). The pluripotential stem cells possess the ability to renew in addition to the capacity to differentiate. It is now clear that bone marrow also contains mesenchymal stem cells which can give rise to connective tissues such as fat cells, fibroblasts, bone and cartilage. The development and preferential survival of a malignant clone of haemopoietic cells, derived from mutated bone marrow stem cells, explains the pathological features of the leukaemias and myelodysplastic syndromes.
If human bone marrow is infused intravenously into a subject without functioning marrow, as during bone marrow transplantation treatment, normal blood cell production returns after a period of several weeks. This finding confirms the presence of pluripotential stem cells in bone marrow and also indicates that the microenvironment of the bone marrow is central to normal blood production; stem cells do not tend to thrive in other sites, and blood production resumes only in the marrow cavities after marrow infusion. Stem cells can be made to circulate in the peripheral blood. This is most conveniently achieved by the administration of one of the cytokines (most commonly G-CSF) responsible for stimulation of haemopoiesis—the colony stimulating factors. Using an extracorporeal centrifugation technique these cells can be harvested and used as an alternative to bone marrow cells in transplantation therapy—a ‘peripheral blood stem cell transplant’. Peripheral blood is used as a source of haemopoietic stem cells more commonly now than bone marrow because engraftment is faster and procurement of peripheral blood stem cells does not require the donor to have a general anaesthetic.
Erythropoiesis
The pronormoblast is the earliest red cell precursor that can be identified in the bone marrow. It is a large cell with prominent nucleoli within the nucleus. By a series of four cell divisions a fully haemoglobinised, non-nucleated erythrocyte is produced. During differentiation the nucleus becomes increasingly condensed and the cytoplasm contains increasing amounts of haemoglobin and less RNA; the early, intermediate and late normoblasts can be distinguished morphologically through the increasing haemoglobin content and progressive nuclear condensation (Fig. 23.12). The nucleus is eventually extruded, leaving a ‘polychromatic’ erythrocyte which remains in the marrow for a further 48 hours; it then circulates for approximately 48 hours before maturing in the spleen to an erythrocyte.
Leukopoiesis
The normal bone marrow contains many more myeloid than nucleated erythroid cells (around 5:1). In the granulocyte series these include the myeloblast, promyelocyte and myelocyte, which are capable of cell division, and the metamyelocyte and band cell, which are undergoing maturation without further division (Fig. 23.12). Maturation of granulocyte precursors involves a reduction in cell size, development of cytoplasmic granules, increased condensation of nuclear chromatin, and irregularity of nuclear outline.
Megakaryocytopoiesis
Megakaryocyte maturation is unique. The earliest identifiable precursor, the megakaryoblast, is a large cell that undergoes nuclear replication without cell division, the cytoplasmic volume increasing as the nuclear material increases, in multiples of 2, up to 32N (Fig. 23.12). Cytoplasmic maturation occurs, often at the 8N stage, and platelets are released. Megakaryocytes are not seen in peripheral blood by routine methods.
Blood cell kinetics
Platelets circulate for approximately 10 days. The spleen acts as a reservoir of platelets; some 30% are present in the spleen at any time.
Haemoglobin
Structure, synthesis and metabolism
By 1 month of age red cell precursors synthesise predominantly haemoglobin A, composed of four haem groups and four polypeptide (globin) chains (Fig. 23.13), of which two molecular forms are present: alpha and beta chains. Haemoglobin A thus has the structure α2β2. Up to 2.5% of the haemoglobin in adults has delta chains (α2δ2)—haemoglobin A2; and up to 1% of the haemoglobin in adults has gamma chains (α2γ2)—haemoglobin F. Adult blood therefore has predominantly haemoglobin A with some A2 and F (Table 23.3).
In later fetal and early neonatal life haemoglobin F predominates. In early fetal life three other haemoglobins are present: Gower 1, Gower 2 and Portland (Table 23.3).
The whole haemoglobin molecule is thus composed of a tetramer of globin chains, each with a haem group. The complex structure of the molecule is responsible for its oxygen (O2) binding characteristics, the globin chains moving against each other during transfer of O2. The affinity of the haemoglobin molecule for O2 is also controlled by its ability to bind the metabolite 2,3-biphosphoglycerate (2,3-BPG) produced during anaerobic respiration. When 2,3-BPG enters the haemoglobin molecule as the beta chains pull apart during release of O2, the affinity for O2 of the haemoglobin–2,3-BPG complex is reduced, allowing O2 to be given up more readily. Haemoglobin F cannot bind 2,3-BPG and thus has a relatively high O2 affinity, facilitating O2 transfer from maternal blood across the placenta.
ANAEMIAS
Anaemia is present when the haemoglobin level falls below around 130g/l in a male or 115g/l in a female. The different lower limits of normal haemoglobin concentration for neonates, infants and children should be noted (Table 23.1).
Classification
Table 23.4 outlines a classification of anaemias. Anaemias are divided into two categories: those where anaemia is due to failure to produce erythrocytes, and those in which erythrocyte loss is increased but production is normal (or usually increased, in response to the anaemia). While useful, this categorisation is an oversimplification, as both mechanisms are present in some anaemias. Thus, in the megaloblastic states, cell production is defective due to lack of vitamin B12 or folic acid for nucleic acid synthesis but, in addition, the erythrocytes that are produced are abnormal and of diminished survival. In thalassaemia, cell production is not optimal due to abnormal haemoglobin synthesis, and there is also increased erythrocyte destruction.
Table 23.4 A classification of anaemias
Type | Cause |
---|---|
Production failure anaemia | |
Haematinic deficiency | Insufficiency of iron, vitamin B12 or folic acid |
Anaemia of chronic disorders | Infection, inflammation and neoplasia |
Dyserythropoiesis | |
Hypoplasia | |
Marrow infiltration | |
Increased red cell loss, lysis or pooling | |
Haemolysis due toabnormality outsidethe red cell | |
Hypersplenism |
PRODUCTION FAILURE ANAEMIAS
The most commonly encountered anaemias are in the production failure group.
Haematinic deficiency
Iron deficiency
Iron metabolism
Iron is an essential requirement. It is also one of the commonest elements present in the Earth’s crust. Excessive iron deposited in the tissues is, however, toxic, causing damage to the myocardium, pancreas and liver in particular (Ch. 16). As the body has no active method for iron excretion, iron status is controlled largely by its absorption; the capacity to absorb iron is, however, limited and any tendency to increased loss of iron, due to haemorrhage, is highly likely to result in a negative iron balance and iron deficiency. These factors explain the high prevalence of iron deficiency.
Ferritin is water soluble and not visible by light microscopy; haemosiderin is insoluble and forms yellow granules. When exposed to potassium ferrocyanide (Perls’ stain) the granules are blue–black. Examination of aspirated bone marrow stained with Perls’ stain can therefore be used to assess body iron stores reliably. When iron stores are normal, haemosiderin is visible, mainly in the reticulo-endothelial cells of the bone marrow. In iron overload, most of the iron is in the form of haemosiderin and can be easily identified.
Mechanisms controlling the rate of iron absorption are becoming better understood. Major influences are the total body iron stores and rate of erythropoiesis. Thus, if iron stores are replete a smaller proportion of available iron is absorbed; when erythropoiesis is more active, due to premature red cell destruction for example, extra iron is absorbed even though total stores may be high. This is a feature in thalassaemia, and iron overload may ensue. A major regulator of iron balance is the liver protein hepcidin. Iron loading leads to rapid production of hepcidin by the liver which, in turn, inhibits intestinal iron absorption and movement of iron from stores. This is achieved by hepcidin downregulating the plasma membrane transfer protein ferroportin. Iron is consequently trapped in iron-exporting cells, including duodenal enterocytes. Plasma iron levels subsequently fall. Conversely, low iron levels lead to downregulation of liver hepcidin and increased iron transfer from the gut and iron-exporting cells so that plasma iron levels rise (Fig. 23.14).
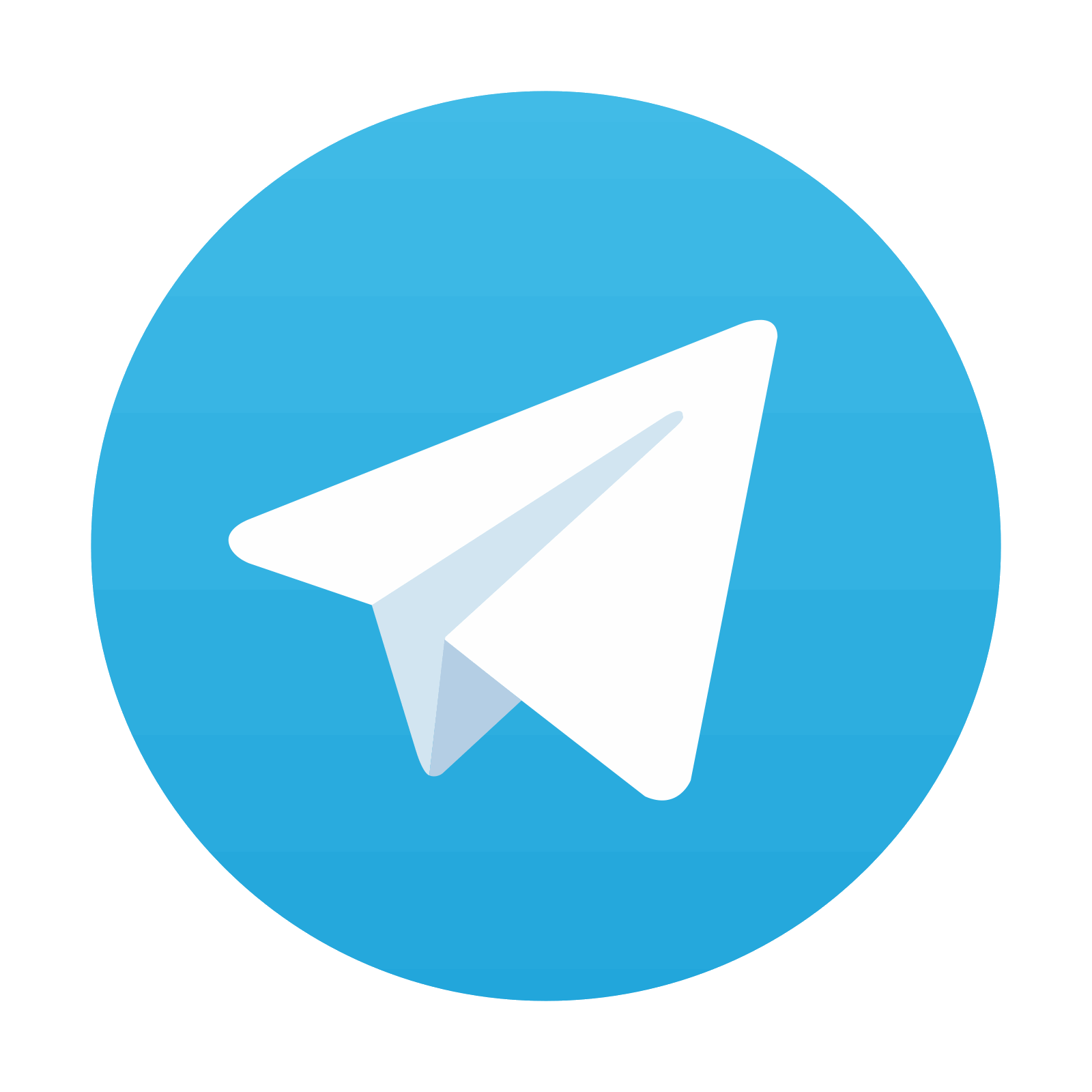
Stay updated, free articles. Join our Telegram channel
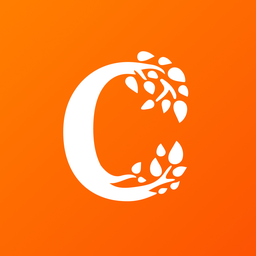
Full access? Get Clinical Tree
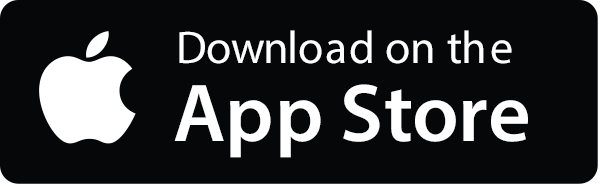
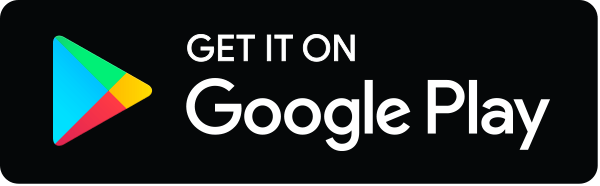
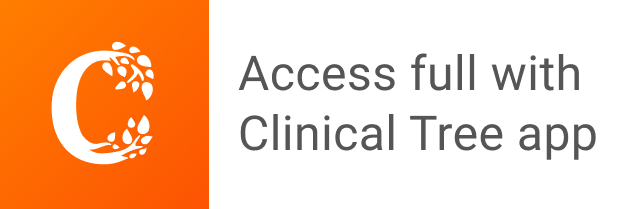