8 Kelly P. Smith1, Maria Borowski1, and Joseph C. Laning2 1Department of Cell and Developmental Biology, University of Massachusetts Medical School, USA 2Research and Development, Provia Laboratories, LLC, USA Since the pioneering studies on nuclear transplantation by John Gurdon [1] and the identification of bone-marrow stem cells by James Till and Ernest McCulloch [2], the last 50+ years have seen remarkable progress in stem-cell science (Figure 8.1). Adult stem cells have been isolated and cultured from numerous tissues and pluripotent stem cells from early embryos (embryonic stem cells, ESCs) have been successfully isolated, cultured, and differentiated into many different cell types. These breakthroughs have allowed researchers to delve into the molecular mechanisms and pathways unique to stem cells that control their ability to develop into numerous cell types. Such fundamental studies have resulted in the current wave of technical innovations that has allowed scientists to “reprogram” adult somatic cells to a state of pluripotency similar to ESCs (induced pluripotent stem cells, iPSCs) [3] or to convert cells from one type to another (direct lineage conversion or transdifferentiation) [4]. These reprogramming technologies are transforming biomedical science, from providing tools for the study of development and disease, to enabling the creation of patient-specific cells for the treatment of a broad range of conditions. Figure 8.1 Stem cell research timeline As an organism develops, stem cells gradually lose their differentiation capacity. The fertilized egg (zygote) and the blastomeres of the four cell-stage morula are totipotent: capable of developing into any embryonic or extraembryonic cell type [5]. Cells making up the blastocyst inner cell mass (ICM) are pluripotent, with the ability to differentiate into any of over 200 cell types found in the mature organism. Beyond this stage, fetal and adult stem cells become more lineage-restricted (multipotent) in their differentiation capacity. The final stem-cell stage is the development of precursor cells, which can change into a very limited set of related terminally differentiated somatic cell types. In addition to their capacity for differentiation, pluripotent cells are capable of unlimited growth. These characteristics allow pluripotent cells to be expanded indefinitely and differentiated to create large quantities of any cell type. This capability makes pluripotent cells an enormously important tool. Cells can be followed through the differentiation process, allowing researchers to investigate development, in both normal and disease states, in new ways. Additionally, the creation of large quantities of specific cell types has applications ranging from drug and toxicity screening to cellular therapy and tissue engineering. Embryonal carcinoma (EC) cells are derived from teratocarcinomas or malignant germ-cell tumors. These tumors possess a mixture of cell types representing all three germ layers as well as malignant undifferentiated EC cells. EC cells extracted from these tumors have been shown to have the capacity to self-renew and differentiate into multiple cell lineages, establishing the concept of the pluripotent cell [6]. EC cell lines were subsequently derived from mouse [7] and human [8]. Although considered pluripotent, EC cells are malignant in the undifferentiated state, are often karyotypically abnormal [9], and have a limited capacity to differentiate [10]. Nonetheless, study of the characteristics of EC cells, how they are cultured, how mouse and human EC cells differ, and how they differentiate has laid the foundation for subsequent advances in stem-cell and developmental biology. ESCs are derived by the isolation of cells from the ICM of a blastocyst-stage embryo and adapting them to in vitro growth. ESCs from mouse embryos were first successfully isolated and cultured in 1981 [11,12]. These mouse ESCs were shown to have the capacity to differentiate into the three germ lineages in vitro by embryoid body formation and to form teratomas when injected into mice. More importantly, pluripotency of these cells was established by their ability to form germ-line chimeras when reintroduced into a mouse blastocyst embryo [13]. The most definitive demonstration of pluripotency was done by injection of mouse ESCs into tetraploid (4N) blastocysts, which are incapable of normal development. This system allows the generation of mice derived completely from the injected ESCs [14,15]. It was not until 1998 that human embryonic stem cells (hESCs) were successfully derived [16]. Although hESCs are similar to mouse embryonic stem cells (mESCs) in many respects, they differ in colony morphology and growth conditions. hESCs differentiate into three germ layers through embryoid body formation [17] and in teratomas [16], but ethical constraints make chimera and tetraploid complementation studies impossible. As discussed later, the true state of pluripotency in hESCs has been the subject of considerable study. Embryonic germ cells (EGCs) are derived from the primordial germ cells (PGCs) found in fetal gonads. PGCs are unipotent, in that they are progenitors of sperm and egg cells via meiosis. These cells cannot self-renew or survive in culture under standard conditions. However, under specific growth conditions mouse [18,19] and human [20,21] EGCs have been derived from these primordial cells. Unlike PGCs, EGCs are capable of self-renewal and of forming cell types representing the three germ layers. However, EGCs are difficult to maintain in an undifferentiated state in culture and spontaneously form embryoid bodies. Unlike other pluripotent cells, they cannot form teratomas in nude mice and have a gene-expression signature that is distinct from other pluripotent cell types [22]. EGCs are morphologically similar to mESCs and express the stem-cell marker SSEA-1, which is expressed in mESCs but not hESCs. Thus, it has been suggested that EGCs may exist in some unique or intermediate state of pluripotency. The plasticity of differentiated cells is evident from cell-fusion studies [23]. In 1974, it was shown that fusion of embryonic carcinoma cells and thymocytes resulted in hybrid cells in which the pluripotent phenotype was maintained [24]. Similarly, hESCs have been fused with human fibroblasts to derive stable tetraploid hybrids that retain the pluripotent characteristics of the ESCs [25]. Cell-fusion hybrids are used primarily to investigate the mechanisms of reprogramming and pluripotency. Somatic cell nuclear transfer (SCNT) involves the transfer of a somatic cell nucleus into an unfertilized oocyte. First demonstrated as a method for cloning animals [26], this technique also offered the prospect of deriving patient-specific pluripotent cells by isolating them from the embryo developed from the hybrid SCNT egg. Despite this prospect, fears surrounding reproductive cloning and the legal and social issues around egg donation have slowed progress in this area. Nonetheless, the reprogramming of human somatic cells to pluripotency by nuclear transfer into an oocyte was recently reported, demonstrating that this technique can be used to produce human pluripotent cells [27]. Groundbreaking work first done in mice by Takahashi and Yamanaka [28] established that differentiated fibroblast cells taken from an adult mouse could be reverted to a pluripotent-like state by introduction of specific transcription factors. In this remarkable study, by homologous recombination β-galactosidase and neomycin resistance genes were incorporated into the Fbx16 gene, which is a nonessential gene expressed in pluripotent stem cells. Using retroviruses, the authors introduced and expressed various combinations of a set of 24 candidate genes identified based on their roles in the maintenance of ESC pluripotency. The result of this inquiry showed that the introduction of four specific transgenes, Oct4, Sox2, Klf4, and c-Myc, could induce fibroblasts to convert to a state similar to ESCs. This result was closely followed by the derivation of human induced pluripotent stem cells (hiPSCs) [29,30]. Since this landmark study, the methods used to reprogram cells have expanded greatly, to include different viruses, episomal delivery, plasmids, proteins, mRNA, miRNAs, and small molecules, as described later. Pluripotency is a complex, dynamic state that has proven difficult to precisely define and measure [31]. Pluripotent cells follow a program of self-renewal while primed to begin a differentiation program in response to specific developmental signals. As such, this state is fairly unstable and there is significant variability in differentiation potential between different pluripotent cell lines [32–34]. Furthermore, it has been found that the pluripotent states of individual ESCs within a culture can vary [35,36]. It has been suggested that such variation in pluripotent states could be an integral mechanism of ESCs, where self-renewal is maintained within the colony or embryo while opportunities for differentiation are explored [37]. Recent studies have uncovered that there are at least two distinct states of pluripotency: the naïve and the primed states, which differ in their epigenetic status and differentiation capacity [38]. Although hESC and mESC are both derived from pre-implantation blastocysts, differences between the two cells in culture are evident. mESCs grow in tightly packed, three-dimensional colonies and have a relatively short doubling time (16 hours); hESCs grow in flatter colonies and their doubling time is more than twice as long (36 hours), and they are maintained with different growth-factor signaling conditions. Furthermore, while mESCs can be passaged as single cells, hESCs must be passaged as clumps, making procedures that require clonal selection, such as genetic modification, much more difficult. Finally, while female hESCs exhibit inactivation of one X chromosome, in mESCs both X chromosomes are active. This indicates that mESCs may be at a different epigenetic stage than hESCs. Other studies have isolated mouse epiblast stem cells (epiSCs) from post-implantation embryos that have characteristics much more similar to hESCs than to mESCs [39,40]. The gold-standard in vivo functional assay for pluripotency is the development of chimeras by reintroduction of ESCs into an embryo where the ESCs contribute to all cell types in the resulting organism. Interestingly, while mESCs pass this functional test, epiSCs and nonhuman primate ESCs [41] have limited capacity for chimera formation. Thus, although all of these cell types exhibit pluripotency in vitro, it has been suggested that mESCs exist in a more potent “naïve” state, while epiSCs and hESCs are in a more “primed” pluripotent state [38, 42]. More recent studies suggest that pluripotency is a metastable state which can be determined by culture and derivation conditions [43,44] and that hESCs can be derived in conditions [45] or exposed to culture conditions post-derivation [46] that allow them to exhibit functional and molecular characteristics similar to those of mESCs. These differences in pluripotent state are important in that they may influence the differentiation capacity and susceptibility to genetic modification of an ESC or iPSC line. Demonstration of the pluripotency of ESC lines when they are derived and during continued expansion of the cultures is necessary to ensure that they retain the capacity for differentiation while spontaneous differentiation is inhibited. Determination of pluripotency in iPSCs is especially important as variability in methodologies, reagents, starting materials, and environmental conditions can lead to the development of lines that are not fully reprogrammed [28, 47]. There are a number of methods that are used to determine pluripotency in a cell line (Table 8.1). Molecular methods can be used to detect expression of genes known to be expressed in pluripotent cells or to define methylation patterns specific to pluripotent cells. Other techniques demonstrate the cells’ capacity to differentiate into specific cell types in vitro or in vivo. While the production of viable adult organisms by the introduction of pluripotent cells into an embryo for chimera formation [48] or by tetraploid (4N) complementation [14,15] may be the most definitive test of pluripotency, this method is clearly not ethical for studies of human pluripotent cells. Table 8.1 Methods of assessing pluripotency The pluripotent state/s is/are reliant upon a number of interconnected processes, including the cellular environment, growth-factor signaling, transcription-factor networks, noncoding RNAs, and epigenetic modifications [49,50]. Current models propose that pluripotency is primarily controlled by the core transcription factors Oct4, Sox2, and Nanog [51]. Oct4 is considered the master regulator of the development and maintenance of pluripotent cells, as Oct4-deficient mouse embryos fail to produce an ICM [52] and suppression of Oct4 in ESCs leads to differentiation along a trophectodermal lineage [53]. Oct4 often forms a heterodimer with Sox2 to bind to the regulatory elements of many different target genes in ESCs [54,55] and promotes an undifferentiated state in ESCs [56,57]. Nanog, Oct4, and Sox2 are reported to co-occupy many target genes in hESCs and function in an interconnected autoregulatory loop to regulate their own expression (Figure 8.2) [51, 53]. These factors also interact with an extended network of other transcription factors to create a regulatory web that promotes pluripotency [58,59]. Figure 8.2 The pluripotency network Interestingly, while these factors bind regulatory sequences to promote transcription, each may also be involved in lineage specification and the repression of lineage-specific genes in ESCs [49, 60]. Overexpression of each of these factors drives differentiation into specific lineages, while knockdown of each factor blocks the same lineage differentiation. While all three factors often co-occupy the regulatory elements of active genes in ESCs, individual factors are frequently bound to repressed loci. Furthermore, active genes are most often bound by multiple factors, while repressed genes are often associated with a single transcription factor [58,59]. Core transcription factors have been found to co-occupy repressed loci encoding important developmental regulators with the polycomb repressive complex 2 (PRC2) [61,62]. PRC2 catalyzes the trimethylation of histone H3 at lysine 27 (H3K27me3) to promote gene silencing [63]. Many of these developmental genes exist within “bivalent domains” in the genome, where genes possess histone modifications that promote gene silencing (HK27me3), but reside within larger domains that have histone modifications of active chromatin (H3K4me3) [64]. Such bivalent modifications are thought to allow the important developmental regulators to exist in a “primed” state in ESCs, where RNA polymerase II is loaded on to the promoter but is held in check by the repressive chromatin [65,66]. In this state, transcription of lineage-specific regulatory genes can be rapidly activated in response to specific developmental cues. Another component of the pluripotency control circuitry involves noncoding RNAs. MicroRNAs (miRNAs) are small noncoding RNAs that regulate gene expression by binding to target mRNAs and facilitating their degradation. Numerous miRNAs have been identified as being expressed in ESCs, and the expression some of these appears to be regulated by binding of the core transcription factors to their gene promoters [67]. Lineage-specific miRNA genes are also bound by the core factors, but are not expressed due to the presence of polycomb repressor complexes such as PRC2, as observed for lineage-specific, protein-coding genes. In turn, several lineage-specific miRNAs can downregulate expression of the core transcription factors [68], as well as components of polycomb group complexes (Figure 8.2) [62]. The functions of large intergenic noncoding RNAs (lincRNAs) are not well understood, but these evolutionarily conserved RNAs interact with chromatin-modifying complexes and influence gene expression [69]. It has been suggested that the lincRNAs could function as flexible scaffolds, bringing together different protein complexes to regulate gene expression. Numerous ESC-specific lincRNAs have been identified whose expression is regulated by core pluripotency factors [70]. Many of these lincRNAs appear to help maintain the pluripotent state and repress expression of lineage-specific gene expression. LincRNAs may facilitate this repression by binding to chromatin-modifying complexes such as PRC2. The variety of cell types in the adult are created via differentiation. Differentiation is a highly complex and regulated process controlled by transcription-factor networks and epigenetic modification of the genome. Epigenetic modifications to chromatin are carried out by a number of different mechanisms, including DNA methylation, post-translational modification of histones, incorporation of histone variants, noncoding RNAs, RNA interference (RNAi, and ATP-dependent chromatin remodeling [71]. ESCs typically have an open euchromatic chromatin state with little inactive heterochromatin. As these cells undergo differentiation, the epigenetic “landscape” of the chromatin is modified [72]. Such modifications occur in very specific patterns throughout the genome, resulting in regions of DNA that are expressed or repressed, and are unique to a particular cell type. Epigenetic modifications are inherited by successive generations of the cells, ensuring that the cellular specialization is maintained. Chromatin remodeling, particularly in the bivalent domains corresponding to important transcription-factor genes, is an essential temporal regulatory switch in the reprogramming process [47, 64, 73–77]. The work of Yamanaka’s group highlighted an underappreciated aspect of genomics and epigenetic control: the extraordinary plasticity of the mammalian genome to reshape itself as part of the reprogramming process. A vital component in the reprogramming timeline is the resetting of the epigenetic landscape. Stochastic remodeling events occur as a result of transcription-factor overexpression leading to endogenous expression of stem-cell factors required for stable cell-line progression [77,78]. Immediately upon forced expression of the transfected reprogramming factors, some proportion of cells starts to divide more quickly and continues down a path of directed somatic gene expression downregulation. However, only a subset of cells continue to upregulate the pluripotent expression profile, and this extended expression is essential in the development of a fully reprogrammed cell line [79,80]. Finally, continuous endogenous pluripotency gene expression establishes a stable ESC-like genomic profile that allows for a sustained functional stem-cell phenotype. This stepwise and multistage process involves so many interconnected pathways and mechanisms that it is not surprising that the path to induced pluripotency is an inefficient one. While several reports have indicated that in most respects human iPSCs and ESCs are globally quite similar, the true depth of the similarity is still being debated at the molecular level. It appears that as analyses become more sophisticated and the detail becomes more refined, the general similarities between ESCs and iPSCs are considerably eroded [3, 76, 81–83]. As with many experimental systems, much of the variability may be generated from differences between investigators relating to cell-culture methods, starting cell populations, reprogramming methods, and screening or characterization techniques. Being different is not necessarily bad, but it does speak to the need for standards and controls by which both research and therapeutic cell lines can be measured before meaningful comparisons can be made. Stem cells, particularly iPSCs, are widely lauded as powerful tools in the fields of regenerative medicine and the study of developmental biology and disease. However, reprogramming of the epigenome is an inexact process and incomplete reprogramming can result in some of the identity or “epigenetic memory” of the source cell being retained during iPSC derivation [84–86]. This memory effect can influence the differentiation capacity of an iPSC line. However, it has been shown that epigenetic memory can be partially erased via extended culture and that DNA methylation inhibitors can significantly influence the differentiation paths of iPSC lines [82, 85, 87]. Whether epigenetic memory is perceived as a liability or an asset likely depends on the intended use of the reprogrammed cell product. Screening for epigenetic memory through whole-genome sequencing may allow for targeted application of specific cell lines for drug screens or in those cell therapies most suited to the differentiative predisposition of the cell line. On the other hand, one might envision the most completely reprogrammed cell lines being reserved for developmental biology investigations requiring unhindered plasticity. In either case, epigenetic memory might be viewed as an advantageous attribute when developing future tools or therapies. The advent of cellular reprogramming has demonstrably changed the landscape of stem-cell biology, disease modeling, and potentially even cellular therapies and personalized medicine. Simply stated, cellular reprogramming is the forced expression of several pathway-specific transcription factors in an adult somatic cell such that the cellular machinery and epigenetic profile revert to a more primitive stem-cell state. The landmark publication in 2006 of the work of Takahashi and Yamanaka found that the genes they screened (Oct3/4, Sox2, Klf4, and c-Myc) could be combined through retroviral transduction achieving prolonged factor expression in order to produce ESC-like cell lines from mouse somatic cells [28]. Although the delivery of the original four transcription factors through viral transduction remains the most commonly used method to date, work continues to improve the efficiency and ultimate safety of the reprogramming process with an eye toward therapeutics. A portion of this section will be devoted to the creative alternatives being explored to achieve this goal. However, our current review will be limited to the four major pathways represented by the original combination of factors. The four main established transcription factors, Oct4, Sox2, Klf4, and c-Myc (OSKM), represent an interconnected reprogramming cocktail that, when expressed transiently, establishes an embryonic-like environment in the target cell. Once expressed, these factors activate the cell’s own endogenous pluripotency pathways, driving the cell toward a stable pluripotent phenotype. Oct4 (Pou5f1), previously mentioned as the “master regulator”, is well known as a transcription factor required for early embryonic development and for the maintenance of pluripotency in ESCs. In experiments limiting the number of factors used to generate iPSC lines, Oct4 was found to be essential to the occurrence of complete reprogramming [88–90]. Sox2, a transcription factor in the SRY-related HMG-box family, is directly involved in the determination of defined lineage development and the maintenance of the undifferentiated state for embryonic and neural stem cells (NSCs). Oct4 and Sox2 can work cooperatively using Sox-Oct motifs that are frequently found within the regulatory sequences of other important pluripotency genes [54]. The expression of many pluripotency-associated genes, including Lefty1 and Nanog, is regulated by enhancers containing Oct3/4 and Sox2 binding motifs, which highlights the dominant regulatory role Oct4 and Sox2 have in maintaining pluripotency [91,92]. Additionally, Sox2 and Oct4 have been reported to bind to a complex of DNA repair proteins, facilitating the transactivation of Nanog [93]. It has been shown in mouse fibroblasts that Sox1 and Sox3 can replace Sox2, although this adversely affects reprogramming efficiency. Kruppel-like factor 4 (Klf4) is required to establish asymmetrical embryo development, as well as a myriad of roles from gut epithelial polarization to tumorigenesis, immune regulation, and iPSC derivation [94–100]. Interestingly, based on studies by Nakagawa and colleagues in murine cells, Klf2 can substitute for Klf4 in reprogramming-factor combinations. Similarly, Klf1 and Klf5 can also substitute for Klf4, but with significantly lower reprogramming efficiency [88]. DNA-binding studies have revealed several shared targets for Oct4, Sox2, and Klf4 related to the control of pluripotency, including Klf4 and Oct4 co-occupying the Nanog promoter, and in the absence of ecoptopic Klf4 expression, endogenous Klf4 expression is required for the reprogramming of somatic cells [101]. This provides further evidence of the connected functional overlap between Sox2 and Klf4 in the disruption of cellular homeostasis and activation of regulatory networks that define pluripotency. The oncogene c-Myc, originally recognized for its role in Burkitt’s lymphoma through chromosomal translocation, was also identified as a key regulator in the reprogramming platform enabling iPSC derivations. c-Myc is a key regulator of both cell growth and metabolism, playing a role in both transformation and cell-cycle entry [102,103]. Additionally, c-Myc participates in the maintenance of ESC pluripotency via the Lif-Stat3 pathway and can induce global histone acetylation, allowing Oct4 and Sox2 to bind to their specific target loci [104,105]. Alternatively, it has been suggested that c-Myc amplification of existing gene profiles due to increased c-Myc abundance and favorable epigenetic access allows for the vast array of oncogenic effects seen when c-Myc is overexpressed, as is the case under many reprogramming methods [106]. Also, the specific substitution of c-Myc has been used in the successful reprogramming of human cells by replacement of c-Myc with L-Myc or N-Myc [88]. While it is widely considered that Myc, in any form, is important for efficient reprogramming, it has been shown that it is not required for reprogramming of mouse and human fibroblasts [30, 88, 107]. However, subsequent studies have shown that adding back c-Myc significantly augments reprogramming efficiency [108]. Numerous studies continue to explore new twists to the original four-factor combination. For instance, reprogramming has been demonstrated using only Oct4 and Sox2 [109]. Furthermore, Scholer and his colleagues [110] have demonstrated that Oct4 alone was able to convert human NSCs into iPSCs. This strongly suggests a role for the differentiative state of the reprogramming target cell type in the overall ease and efficiency with which a somatic cell converts to an iPSC. Another recent discovery has shown that although Oct4 is essential, it can be replaced with a nuclear receptor gene, Nr5a2, in the derivation of iPSCs from mouse somatic cells [111]. In addition, including the retinoic acid receptor (RARγ) and Nr5a2 with the four factors has been shown to greatly increase reprogramming efficiency in both mouse and human cells [112]. While these and other studies show several compelling factor combinations or reductions can be employed in the reprogramming, the primary set of factors still remains the minimally sufficient combination for efficient complete reprogramming, whether that occurs as a result of the expression of the transcription factors alone or in combination with compounds that substitute for one or more of them. Of course, the process remains influenced by the endogenous expression of the core factors or related pathways, as well as the characteristics of the target cell, such as proliferation rate [113]. The stoichiometry and timing of transcription-factor expression significantly influence the properties of the reprogrammed cells [114] and numerous studies have investigated the temporal and stoichiometric parameters related to one or more of the original reprogramming factors, as well as the potential replacement of certain factors with proteins or small molecules [100]. More recently, studies employing a tagging system using monoclonal antibodies that could be used to analyze the expression levels of the four reprogramming factors in sorted iPSCs found that the most effective ratio (Oct3/4-↑, Sox2-↓, Klf4-↑, c-Myc-↑) was almost 100× more efficient at producing iPSCs than the least optimal ratio (Oct3/4-↓, Sox2-↑, Klf4-↓, c-Myc-↓) [115]. In addition, Tiemann et al. [116] tested 16 different stoichiometric ratios of the four factors and found that only seven combinations were successful in generating any reprogrammed colonies, suggesting that appropriate sustained levels of reprogramming factor expression are required to fully reprogram. Similarly, the length of reprogramming-factor expression is equally important. It has been shown that sustained expression for 12–16 days is needed for complete reprogramming of mouse fibroblasts to pluripotency [117]. This type of information may allow viral, episomal, and protein-based systems to be tailored to the reprogramming cell source type or for the addition of small molecules in order to increase the efficiency of one or more factors and thus achieve the optimal ratio. Based on the transexpression of the prototypical reprogramming factors (OKSM), the importance of the type and origin of the cell to be reprogrammed cannot be overlooked. As with many scientific methods, there is a balance to be struck between ease of material procurement, target-cell reprogramming efficiency in relation to reprogramming method, species, and intended outcome or use of the reprogrammed cell line. Keratinocytes and peripheral blood cell types, such as T cells, are readily available and reasonably receptive to reprogramming irrespective of reprogramming method. Fibroblasts are equally easy to acquire, yet are as much as 100× less efficient in yielding iPSC lines than keratinocytes using a viral OKSM methodology [118]. The availability of cord blood as a reprogramming source material has the added advantage of potentially only requiring Oct4 and Sox2 to reprogram to iPSC, due in part to the relatively high proportion of proliferative progenitor phenotypes found in cord blood [119]. For some disease-specific iPSC derivation strategies, blood samples may be the most readily available source material. However, numerous biobanks are beginning to archive and make available samples from well-characterized familial disease trees based on skin fibroblast lines in order to aid investigators. It is still not entirely clear whether a strict hierarchy can be assigned to any given cell type or reprogramming method with respect to overall efficiency. Alternatively, it may be more helpful to categorize such potential hierarchies in the context of the methods and cell sources available, as well as the intended use of the derived reprogramming products. The potential use as a therapeutic will require significantly different methods than iPSC lines derived for research use, so some situational flexibility will play a role in the final choice of cell sourcing and reprogramming method. Balancing the availability of the cell source with the potential efficiency of reprogramming is not insignificant. Generally speaking, highly specialized terminally differentiated cell types like T lymphocytes and fibroblasts are easy to obtain yet harder to reprogram, while cord blood cells and germline cells are the reverse. While these gross generalizations may be true on the surface, new refinements and à-la-carte strategies for reprogramming may change these beliefs to some extent. Early methods have utilized viral vectors which integrate into the genome to boost the efficiency of reprogramming to pluripotency. However, the potential for insertional mutagenesis and aberrant expression of reprogramming genes embedded in the genome impedes the path of reprogrammed cells to the clinic. So, recent efforts have turned to the development of nonintegrative reprogramming methods, including nonviral episomal plasmid systems, non-DNA methods, and nonintegrative viral systems with or without reprogramming enhancers [120–124]. The convenience and effectiveness of viral-based reprogramming methods makes them a valuable methodology for preparing iPSCs from numerous cell types from a number of species. While the lack of a “footprint”-free resultant cell line is currently problematic for downstream translational applications of these cells, the method itself, especially with the later iterations that are now widely used, make viral delivery of reprogramming factors for research-directed cell lines a viable and successful approach. Having said that, new nonintegrative viral systems such as adeno-associated virus- or sendai virus-based delivery systems are becoming more popular and replacing the traditional retroviral and lentiviral gene-delivery systems. However, the choice of delivery system is primarily dependent upon the target cell type and species, as well as the intended use of the reprogrammed products. The original retroviral-based reprogramming studies showed both success and ample room for improvement [29]. This work has been confirmed and extended by other groups, which showed that stable genomic integration and high expression of four factors – Oct4/Sox2/Klf4/c-Myc or Oct4/Sox2/Nanog/Lin28 – can reprogram fibroblast cells, B cells, and liver and stomach epithelial cells into iPSCs [30, 125]. The main drawback to the integrative viral methods is the unpredictable and variable nature of the genomic integration of the reprogramming genes, especially when one or more of these factors are known oncogenes. Several genetic-engineering advances have sought to minimize these unforeseen effects through polycistronic single-vector delivery systems and the use of Cre-loxP-expression systems [126,127]. While these systems allow for more controlled integration and virtually complete excision of the reprogramming factors, respectively, neither completely resolves the potential for unforeseen insertional or mutational affects to the host genome. Using nonintegrating adenovirus or sendai virus delivery systems, successful four-factor reprogramming has been performed on human and mouse cells with a variety of cell types [121,122]. These transient expression systems utilize host machinery, both cytoplasmic and nuclear, without integrating into the host genome and, like episomal transfection-based methods, the absence of specific vector sequences can be verified in fully reprogrammed cell lines through standard PCR methods. This is becoming an increasingly sought after quality with respect to the iPSC derivation process as a whole. Adenovirus and adeno-associated viral systems are relatively less efficient than integrating and sendai-based viral delivery systems, but offer increased safety characteristics [128]. The combined bump in efficiency and nonintegrative qualities for the sendai virus delivery system makes it an increasingly popular alternative to standard viral methods [121]. One drawback of this methodology is the current cost of the delivery system, putting large-panel derivations out of the reach of most investigators. Other viral vector systems, such as lentivirus and gamma-retrovirus, require integration into the host genome for replication and can therefore disrupt the genomic integrity of the cells that are reprogrammed, rendering iPSCs and their derivatives less safe for clinical applications or altering gene-expression patterns, which can compromise compound screens or disease pathway analyses. Overall, the classic lentiviral or retroviral systems and more sophisticated constructs allowing selective removal of viral sequences still represent one of the most efficient and economical methodologies for reprogramming somatic cells of all types. Clearly, the integrating viral delivery methods can be upwards of 1000× more efficient at generating fully reprogrammed cell lines (Table 8.2), but these and other factors must be weighed when choosing a reprogramming methodology. It has been shown that residual expression of reprogramming factors can affect the transcriptional machinery and epigenetic profile of reprogrammed cells and should be factored into the overall cell-line development strategy [140–142]. Table 8.2 Derivation methods for reprogramming CBP, cord blood progenitors; HFF, human foreskin fibroblasts; MEF, mouse embryonic fibroblasts; HDF, human dermal fibroblasts; LEC, liver epithelial cells; GEC, gastrointestinal epithelial cells; MSC, mesenchymal stem cells; HNF, human neonatal fibroblasts; HK, human keratinocytes; PBL, peripheral blood leukocytes. An economical and yet relatively inefficient choice for somatic cell reprogramming is the use of nucleic acid in either linearized-DNA or episomal-vector formats [120, 132, 133, 143]. This method allows for the generation of iPSC lines that are free of vector or transgene sequences and have been used to reprogram a variety of somatic cell types. More recently, episomal reprogramming has been used in conjunction with a cocktail of small molecules to increase efficiencies 6–10-fold over episomal vector alone [144]. Serial transfection of multiple or single polycistronic plasmids is required for even modestly efficient reprogramming for most vector systems. Yu et al. [144] designed an Epstein–Barr virus nuclear antigen EBNA-containing episome that is self-replicating, obviating the need for multiple transfections and certainly reducing cell loss due to transfection toxicity. However, this nonintegrative episomal delivery system does not guarantee complete lack of plasmid integration and may require extensive dilution through cell replication to remove all instances of plasmid sequences [145]. Several important parameters govern success in episomal reprogramming, including but not limited to: (i) transfection method and efficiency; (ii) rapid dilution of transfected vector due to highly proliferative target cells; (iii) active silencing of plasmid sequences through host methylation; (iv) target cell type; and (v) the overall size of the minicircle vector [128]. In all episomal reprogramming methods, proper post-reprogramming screening should include molecular methods for the detection of vector sequences. Episomal delivery offers significant advantages over other methods, including an increased safety profile and compatibility with any number of external factors/enhancers that enable the tailoring of the method to research or therapeutic development requirements. A significant shift in nucleic acid-based reprogramming came in 2010 when Rossi and colleagues published on the highly efficient use of synthetic mRNA species to orchestrate reprogramming in multiple human cell types [123]. This method of using synthetic mRNA species to drive exogenous expression of the four canonic Yamanaka reprogramming factors proved to be a reliable (up to 10000× more efficient than standard episomal reprograming systems; see Table 8.2) defined method that gives rise to footprint-free iPSC lines. One drawback is the repeated transfections required over 12–16 days to ensure sufficiently stable factor expression to give rise to completely reprogrammed cell lines. Some recent effort has been devoted to qualitative changes in mRNA design, delivery, and screening methods that can reduce this production time, but the method still requires daily transfections for an extended period of time [146]. It is suggested that the initial investment in reagents and effort with the mRNA method can be recouped in the high frequency of fully reprogrammed colonies resulting from the method, thereby saving on screening time. As reprogramming methods push toward solutions enabling clinical use of iPSC lines derived from patients as personalized medicine, it is clear that nonintegrative methods like mRNA are setting the pace. miRNAs could be as instrumental in reprogramming as the transcription factors or epigenetic regulators that are so well known as drivers of somatic cell reprogramming. miRNAs are known to be key regulators of cell fate in ESCs, the development of the blastocyst, and various differentiation pathways [147–151]. Therefore, it can easily be envisioned that miRNAs could be utilized to impart cell-fate decisions in somatic reprogramming through the activation and repression of lineage-specific genes. The use of miRNAs as adjuvants to reprogramming through the addition of selected miRNA species to episomal or viral vector systems may prove useful. The transcription factor OSK plus miR-291-3p, miR-294, or miR-295 has been shown to consistently increase the number of Oct4- GFP+ colonies as compared to controls transduced with OSK alone. The miR-294 mimic showed the greatest effects, increasing efficiency by up to 10-fold for transduced mouse embryonic fibroblasts (MEFs) [152]. Miyoshi et al. [138] showed that the transfection of a combination of mir-200c, mir-302s, and mir-369s could reprogram both mouse and human somatic cells. It has recently been shown that the mechanism by which sodium butyrate enhances hiPSC reprogramming efficiency works through the induction of the miR302/367 miRNA cluster [153]. The blending of traditional methodologies for reprogramming and miRNAs may prove a powerful combination and afford researchers selective and increasingly less invasive methods by which to generate iPSCs [154]. Protein-based delivery of reprogramming factors has been demonstrated, but it has extremely slow kinetics, requiring significant time investment – as much as 6 weeks [124]. Another drawback to the use of proteins as factor-delivery modules is that transmembrane delivery can be challenging and therefore intracellular localization is very inefficient [155]. Amino acid segments from HIV-TAT and other basic peptides (CPPs) can significantly increase membrane translocation and allow the delivery of nucleic acid or protein elements to the nucleus [156,157]. Improvements in the targeting or stability of engineered proteins may make these systems more effective in the future; however, current requirements for numerous applications of the reprogramming cocktail over many weeks hampers mainstream usage of such systems. Several studies have shown that epigenetic remodeling is a rate-limiting step in reprogramming [109, 153, 158]. As shown in Table 8.3
An Introduction to Cellular Reprogramming: The Plasticity of Cell Fates and Identities
8.1 Defining Cell Potency
8.2 Types of Pluripotent Cell
8.2.1 Isolated Cell Types
8.2.1.1 Embryonal Carcinoma Cells
8.2.1.2 Embryonic Stem Cells
8.2.1.3 Embryonic Germ Cells
8.2.2 Reprogrammed Cell Types
8.2.2.1 Cell-Fusion Hybrids
8.2.2.2 Somatic Cell Nuclear Transfer Cells
8.2.2.3 Induced Pluripotent Stem Cells
8.3 Defining Pluripotency
Analysis of pluripotency
Tests
Methods
Molecular
Expression of pluripotency-specific genes
Immunocytochemical staining (marker protein detection) and/or quantitative RTPCR (mRNA detection)
Whole-genome expression
Microarray hybridization
Epigenetic analysis
Determination of the degree of methylation of ESC-specific gene promoters
In vitro
Embryoid body formation
Spontaneous differentiation of cell clumps followed by detection of lineage-specific markers by ICC or qRTPCR.
Directed differentiation
Differentiation of cultures toward specific cell types
In vivo
Teratoma formation
Spontaneous differentiation of cells injected into specific sites in mice
Chimera production
Introduction of cells into a mouse blastocyst and detection of differentiated progeny of the introduced cells in all tissues of the adult
Tetraploid complementation
Introduction of cells into a mouse 4N (tetraploid) blastocyst. Mature mouse will develop entirely from introduced cells
8.4 The Molecular Basis of Pluripotency
8.5 Cellular Reprogramming: Altering the Epigenetic State
8.6 Cellular Reprogramming: Primary Regulatory Pathways
8.6.1 Temporal and Stoichiometric Considerations
8.6.2 Target Cell Type
8.7 Reprogramming Methods
8.7.1 Viral-Driven
Derivation method
Factors/pathways
Cell types reprogrammed
Main advantages
Efficiency
Reference
Retroviral
OKSM, OSNL, OKS, OS
HDF, HFF, MEF, HK, CBP. PBL, LEC, GEC
Efficient
0.001–0.1%
[28,88,109]
Lentiviral
OKSM, OSNL, OKS
HDF, HFF, MEF, HK, CBP. PBL, LEC, GEC
Efficient
0.001–0.1%
[29,126]
Adenoviral
OKSM, OSNL, OKS?
MEF, LEC, HDF, MSC
Nonintegrative
0.0001–0.01%
[122,129]
Sendai virus
OKSM
PBL, HDF
Efficient, nonintegrative
0.01–1%
[121,130]
Cre-loxP virus
OKSM
HK, HDF
Efficient, reduced genetic footprint
0.01–0.0001%
[99,127,131]
Plasmid/episomal
OKSM
HK, HDF, PBL
Nonintegrative
0.0001%
[132,133]
Plasmid/transposon
OKSM
MEF, HDF
Nonintegrative
0.1–1%
[134,135]
mRNA
OKSM, OKSML
HDF, PBL
Nonintegrative
0.01–1%
[123,136]
Protein
OKSM
HDF, MEF, HNF
Nonintegrative
0.001–0.00001%
[124,137]
miRNA
mir-302, mir-371, mir-367, mir-200c
HDF, PBL
Nonintegrative
0.1–10%
[138,139]
8.7.2 Nucleic Acid/Episomal-Driven
8.7.3 mRNA-Driven
8.7.4 miRNA-Driven
8.7.5 Protein-Driven
8.7.6 External Factors/Enhancers
Stay updated, free articles. Join our Telegram channel
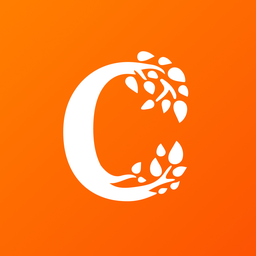
Full access? Get Clinical Tree
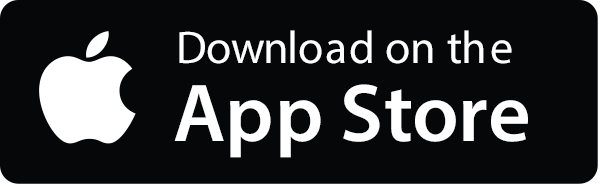
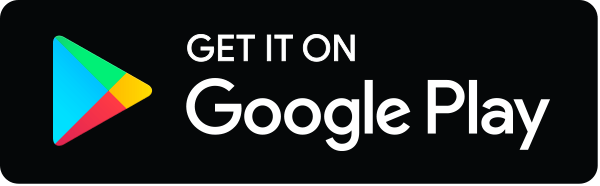