Fig. 2.1
Mechanisms of amyloid formation and toxicity. Intrinsic instability, increased concentration, mutations, proteolytic cleavage or a combination thereof can favour the conversion of the amyloidogenic precursor into its misfolded conformation. This process results in the formation of prefibrillar species and ultimately amyloid fibrils. Oligomers in equilibrium with amyloid fibrils are believed to exert a direct cytotoxic effect. Interactions with tissue factors, including glycosaminoglycans (GAGs) and serum amyloid P component (SAP), contribute to the formation and persistence of amyloid deposits, which contribute to the functional impairment of affected organs
More often, a combination of these factors actually determines the amyloidogenicity of an individual protein:
1.
Increased concentration
Some proteins can form amyloid only at persistently increased concentrations, as is the case for the acute phase reactant serum amyloid A (SAA) in chronic inflammations [7] and for wild-type β2-microglobulin in patients with end-stage renal failure, where kidney-mediated clearance of this protein from the circulation is not efficiently replaced by dialysis [8].
2.
Mutations
Mutations, although frequently consisting of only a single amino acid substitution, can dramatically destabilize a protein and favour its aggregation and subsequent amyloid deposition—as demonstrated for cystatin C [9], transthyretin [10], lysozyme [11], gelsolin [12], apolipoprotein A-I [13] and β2-microglobulin [14]. Such mutations (reported in a dedicated online registry [15], at www.amyloidosismutations.com) are the molecular substrate for a group of conditions that are collectively termed hereditary amyloidoses [16].
3.
Proteolytic cleavage
In the majority of cases, only a limited portion of the amyloidogenic precursor is actually found in amyloid deposits. The enzymes responsible for the proteolytic remodelling of the precursor are largely unknown. They are postulated to be extracellular and whether proteolysis occurs before or after the monomer has been incorporated within the amyloid fibrils is currently a matter of speculation [1]. There have been only a few cases where such enzymes have been unambiguously identified and the proteolysis has been shown to take place before amyloidogenesis: the enzyme furin, residing in the Golgi apparatus, cleaves ABri [17] and gelsolin [18] and the membrane proteases β- and γ-secretases release amyloid-β (Aβ) peptides from the amyloid precursor protein (APP) in Alzheimer’s disease [19]. Remarkably, several mutations in genes encoding APP or presenilins, members of the γ-secretases, can strongly favour the amyloidogenic proteolytic cleavage of APP and are hence associated with familial forms of the disease [20].
4.
Intrinsic instability
There are a few proteins that are believed to display an intrinsic propensity to adopt more than one conformation, a feature strongly influenced by hydrophobicity, electric charge and secondary structure [5] and which might—in the long term—lead to amyloid formation. Typical examples of this class of amyloidogenic proteins are transthyretin and apolipoprotein A-I, both associated, in their wild-type conformation, with ageing-related amyloid deposition [21–24]. The intrinsic instability of both proteins is further increased by pathogenic mutations associated with hereditary forms of the disease [25, 26]. Intriguingly, the propensity of wild-type transthyretin to form amyloid is also enhanced by exposure to a mutant disease-related form of the protein. In individuals heterozygous for one of the pathogenic mutation, both the mutated and the wild-type transthyretin are found in deposits [27–30], and this phenomenon explains why cardiac amyloid deposits can further progress in patients for whom liver transplantation has minimized the production of the mutant protein [27–29].
The inherent amyloidogenicity of a specific protein, per se, is not sufficient to explain the likelihood that amyloid deposition finally occurs in vivo. For example, only a minority of patients with long-lasting inflammation and subsequent elevation of SAA levels develop AA amyloidosis [31, 32]. Similarly, the disease-associated Val30Met mutation of transthyretin shows significant differences in penetrance and clinical presentation among different ethnic groups and geographic areas [33]. Other factors, both environmental and genetic, are probably involved and understanding their roles will certainly improve our current knowledge of amyloidogenesis and, hopefully, pave the way to the discovery of novel therapeutic approaches.
Common Constituents of Amyloid Deposits
Amyloid deposits are classified and named based on the chemical nature of the most abundant fibril protein, according to internationally adopted nomenclature guidelines [34]. However, additional components are regularly found in the deposits (Fig. 2.1), including proteoglycans [35], glycosaminoglycans [36], and the pentraxin family member, serum amyloid P component (SAP) [37]. Proteoglycans and glycosaminoglycans can contribute to the formation and stabilization of amyloid fibrils and, through their interaction with extracellular matrix elements, influence the localization of amyloid deposits [1]. The SAP component binds avidly and reversibly to all types of amyloid [38], a property which allows for the clinical use of a radiolabeled version of this protein for scintigraphic imaging of amyloid deposits [39, 40], and which renders amyloid resistant to degradation [41]. This latter feature, and the observation that mice genetically devoid of SAP show a delayed deposition of experimentally-induced amyloid [42], is the rationale for the development of therapeutic approaches aimed at reducing circulating and amyloid-associated SAP through small palindromic drugs and antibodies [43, 44], which have already started to enter into the clinical phase of testing [44].
Amyloid Structure
Electron microscopy and X-ray diffraction analysis have revealed that amyloid deposits are composed of rigid, non-branching fibrils with an average diameter of 7.5–10 nm and a cross-β super-secondary structure [45–47]. More recently, the application of solid-state nuclear magnetic resonance spectroscopy to large amyloid fibrils [48–50] and the successful preparation of microcrystals of small amyloid-like peptides which can be subjected to X-ray diffraction analysis [51, 52] have enabled refined structural studies of amyloids (reviewed in [3, 5, 53]), sometimes with atomic resolution [54, 55]. These results have corroborated the notion that, despite almost identical morphological and ultrastructural properties, amyloid fibrils do have a certain degree of variation [5], and this will hopefully deepen our understanding of the molecular basis of amyloid diseases.
Amyloid Formation: From Monomers to Fibrils
Self-intuitively, the transition from soluble monomeric and (usually) folded proteins to insoluble multimeric fibrillar aggregates with the above-mentioned structural properties requires drastic modifications of the protein’s original conformation. This is believed to occur mainly through a partial unfolding of the native globular amyloidogenic precursor, or part of it, followed by aggregation of the unfolded moieties to finally form the amyloid fibrils [56, 57]. Said modifications are thermodynamically possible because the aggregation-prone unfolded state of the protein is separated from the native folded state—which corresponds to the minimum content of free energy and therefore to the most stable conformation—by a low energetic barrier which can be easily surmounted via naturally occurring thermal fluctuations [58].
However, alternative mechanisms can also play a role in amyloid fibrillogenesis. Under certain circumstances, globular proteins can oligomerize in a native-like state and only afterwards experience major conformational changes resulting in amyloid fibril formation [58]. This mechanism is reminiscent of the fibrillogenic pathway followed by naturally unfolded proteins and by protein fragments, which are unable to fold correctly when released from the protein of origin [58].
Kinetics of Fibril Formation
In vitro studies have shown that amyloid fibril formation proceeds, in many instances, through a “nucleated growth” mechanism, which is reminiscent of the mechanism of crystallization [5]. Starting from a solution of monomeric proteins, there is an initial phase, termed lag phase, where aggregation does not occur. However, as soon as a critical nucleus has been generated, fibril formation begins and further proceeds with very fast kinetics: any amyloidogenic precursor in its aggregation-prone conformation is rapidly incorporated into the growing fibrils [1, 5, 62]. Moreover, fragmentation of growing fibrils and other nucleation events further accelerate amyloidogenesis [63].
Organ Tropism
In the localized forms of the disease, the site of amyloid deposition is determined by the cellular source of the amyloidogenic precursor: the pancreatic islets for the β-cell derived amylin in type two diabetes, the brain for the neuron-derived Aβ in Alzheimer’s disease, the thyroid gland for the parafollicular cell-derived calcitonin in C-cell thyroid tumours, etc. Conversely, in systemic amyloidoses, the precursor protein circulates in the blood stream and has potential access to almost any organ or tissue in the body. Nonetheless, specific amyloidogenic proteins tend to deposit predominantly in defined organs, for example, the kidney for the fibrinogen Aα chain and leukocyte chemotactic factor 2, the joints for wild-type β2-microglobulin, and the peripheral nerves for the transthyretin Met30 variant [1]. The reasons for the peculiar tropism of some amyloidogenic proteins are not fully known. Several factors could contribute to determining the site of amyloid deposition, including the presence of amyloid seeds, local protein concentration, pH, presence of proteolytic enzymes, interaction with collagen [66], glycosaminoglycans [67] or cellular receptors [1, 68].
Mechanisms of Tissue Damage
The process of amyloid formation and deposition usually results in tissue damage and organ dysfunction through mechanisms that have not been fully elucidated [1]. The presence of large amounts of amyloid material can subvert the tissue architecture and mechanically interfere with the physiologic function of affected organs (Fig. 2.1) [38].
However, compelling evidence now supports the idea that prefibrillar oligomeric species, and not fibrillar amyloid deposits, are the bona fide toxic species in amyloid diseases (Fig. 2.1) [1, 5]. Prefibrillar oligomers from transthyretin [69, 70], Aβ [71–73], immunoglobulin light chains [74–76] and the cellular prion protein [77] have been shown to be toxic in vitro and/or in vivo.
As a consequence of the conformational change underlying their formation, prefibrillar aggregates are expected to expose, at their surface, groups that are normally buried inside the folded proteins or dispersed in the natively unfolded proteins [5]. These structural properties of prefibrillar aggregates are regarded as potential effectors of amyloid toxicity [5] and recent in vitro studies support this hypothesis [78].
Immunoglobulin Light Chain Amyloidosis (AL)
With an overall incidence of 8.9 new cases per million person/year, immunoglobulin light chain (AL) amyloidosis is the most common form of systemic amyloidosis in Western countries [79, 80].
In this disease entity, a plasma cell clone is responsible for the production of monoclonal immunoglobulin light chains, which undergo aggregation and form amyloid deposits either systemically or, rarely, locally [81]. The latter condition is defined as localized AL amyloidosis and accounts for approximately 5 % of all AL cases.
The Amyloidogenic Clone
The plasma cell clone in systemic AL amyloidosis typically resides in the bone marrow, and the infiltrate is generally of modest size (median of bone marrow plasma cells: 9 %). The degree of plasma cell proliferation is usually low or undetectable [82], and less than 1 % of AL patients without multiple myeloma at diagnosis eventually progress to multiple myeloma over time [83]. Indeed, in AL amyloidosis the clinical manifestations are dominated by end-organ damage caused by the amyloidogenic light chains, rather than by the direct tumour burden of the plasma cell clone [81] (Fig. 2.2).


Fig. 2.2
B-cell neoplasia vs. M-component-related diseases. In B-cell neoplasias (MM multiple myeloma, WM Waldenström macroglobulinemia, NHL non-Hodgkin lymphoma and CLL chronic lymphocytic leukemia), the clinical pattern is usually dominated by systemic effects caused by expansion of the malignant clone, whereas the monoclonal protein may cause hyperviscosity syndrome or kidney damage. In less common disorders, termed M-component related disorders (AL immunoglobulin light chain amyloidosis, AH immunoglobulin heavy chain amyloidosis, LCDD light chain deposition disease, LHCDD light and heavy chain deposition disease, HCDD heavy chain deposition disease, GOMMID glomerulonephritis with organized microtubular monoclonal immunoglobulin deposits), the biological effects of the monoclonal protein may account for most of the clinical manifestations and determine the prognosis. There are overlaps between these two groups; for instance, the IgM of a patient with Waldenström macroglobulinemia may have a cold agglutinin activity and a myeloma clone can secrete an amyloidogenic light chain (dashed line). Rarely, M-component-related diseases can progress to an overt B-cell neoplasia (dotted line). (This is a modified version of a figure which was originally published in Blood. Merlini G, Stone MJ: Dangerous small B-cell clones. Blood 108:2520–30, 2006. © The American Society of Hematology)
Exceptionally, AL amyloidosis can arise in association with Waldenström macroglobulinemia [84], light chain deposition disease [85], POEMS syndrome [86, 87], non-Hodgkin lymphoma [88, 89], and chronic lymphocytic leukemia [90].
Besides mature bone marrow plasma cells, the amyloidogenic clone also includes more undifferentiated bone marrow progenitors as well as mature B lymphocytes and plasma cells in peripheral blood [91–94]. Clonal plasma cell elements can also be found in the spleen and might serve as a source of amyloidogenic light chains [95].
Exposure to certain cytokines leads to in vitro differentiation of peripheral clonal elements into plasma cells that are very similar to their bone marrow counterparts [96]. These observations suggest the existence of an intra-clonal differentiation process in AL: circulating elements would serve as precursors that are able to differentiate and sustain the accumulation of bone marrow plasma cells [97].
The degree of bone marrow infiltration and plasma cell clonality, with or without hypercalcemia, renal failure, anemia and lytic bone lesions attributable to clonal expansion of plasma cells (CRAB criteria) [98–100], the percentage of circulating peripheral blood plasma cells [101], serum levels of amyloidogenic free light chains [102–104] and other markers of plasma cell burden [104] are of prognostic value [105].
Clonotypic cells can contaminate apheretic stem cell harvests of AL patients and may contribute to relapse after high-dose chemotherapy followed by stem cell transplantation [93, 106].
The amyloidogenic clone has minimal but measurable kinetics of proliferation and replicating elements are represented by lymphoplasmacytoid cells within the bone marrow [91]. The percentage of bone marrow plasma cells in their DNA synthetic (S) phase of the cell cycle defines the so-called plasma cell labelling index and correlates with a poorer prognosis in AL patients [82].
Amyloidogenic plasma cells frequently display aneuploidy due to numerical chromosomal alterations [107]. Translocations affecting the 14q32 locus of immunoglobulin heavy chains are present in the majority of cases (>75 %) [108]. Particularly frequent are t(11;14)(q13;q32) [108] and t(4;14)(p16.3;q32) [109], present in 55 % and 14 % of cases, respectively. In contrast, hyperdiploidy is relatively uncommon with respect to other plasma cell disorders and is observed in only 11 % of AL cases [110]. Recently, gain of 1q21, which is present in approximately 20 % of AL cases, has been identified as an independent adverse prognostic factor in AL amyloidosis patients treated with standard chemotherapy [111].
Common single nucleotide polymorphisms representing risk alleles for MGUS [112] and multiple myeloma [113, 114] are also associated with AL amyloidosis, highlighting a common genetic susceptibility underlying these conditions [115]. Moreover, gene expression analysis has identified a set of 12 genes—including CCND1, the gene encoding cyclin D1—which can distinguish between AL amyloidosis and multiple myeloma with an accuracy of classification of 92 % [116]. According to this study, amyloidogenic plasma cells display an intermediate pattern of gene expression with respect to normal and myeloma plasma cells. The potential pathophysiological significance of cyclin D1 overexpression in amyloidogenic plasma cells has been the object of further investigations. In particular, cyclin D1 levels were found to be associated with preferential secretion of free light chains only and possibly with response to therapy and overall survival [117]. Moreover, amyloidogenic plasma cells were found to express CD32B [118] and, in a minority of cases, CD20 [119] and CD52 [120], which may be novel molecular targets for anti-AL immunotherapy.
Future studies will have to investigate to which extent chromosomal abnormalities, gene expression patterns or other genetic features of amyloidogenic plasma cells influence response to different chemotherapeutics or other aspects of the disease. The hope is that the increased mechanistic understanding of the biology of amyloidogenic plasma cells could guide therapeutic interventions against these small, yet detrimental clones [81].
Genetics of Amyloidogenic Light Chains
Only a small fraction of monoclonal light chains, believed to be less than 5 %, can form amyloid fibrils in vivo. In a clinical series of 1384 patients with a monoclonal gammopathy of undetermined significance, with an 11,009 person/years follow-up, only ten patients developed AL amyloidosis [121]. Analogously, only 1 % of patients with active multiple myeloma subsequently develop AL amyloidosis [122]. Therefore, the potential to form amyloid fibrils is believed to reside in specific structural features of immunoglobulin light chains. As opposed to other plasma cell disorders, the isotype of amyloidogenic light chains is, in most cases, λ (75 %). Moreover, germ-line gene usage for the variable region of λ light chains in AL amyloidosis differ significantly from the germ-line gene usage observed in polyclonal bone marrow cells under normal conditions [123] due to the restricted usage of a small set of genes in AL [124, 125]. This phenomenon of gene restriction can be explained by the substantial over-representation of just three AL-associated gene segments, IGLV2-14 (previously termed Vλ2), IGLV3-1 (Vλ3r) and IGLV6-57 (Vλ6a), which together encode 60 % of amyloid Vλ regions [123–125]. Of note, light chains belonging to the IGLV6 family are almost invariably associated with AL amyloidosis [125]. On the other hand, germ-line gene usage in κ light chains is less well studied. It seems that a few gene segments (IGKV1 and IGKV3 families) are preferentially used in AL amyloidosis [125].
Recent results from a clinical series of 53 AL patients undergoing stem cell transplantation suggest that clonal variable light chain gene usage might influence global cardiac function and long-term mortality [126]. These preliminary observations, if confirmed, could shed new light on the mechanisms of amyloid toxicity and organ dysfunction.
In AL amyloidosis patients, immunoglobulin light chain variable regions were found to be hypermutated and mutations were not associated with intraclonal diversification within the bone marrow, indicating that amyloidogenic light chains undergo antigen-driven selection [127]. These data suggest that amyloidogenic clones may arise from a neoplastic transformation of differentiated B lymphoid elements selected during antibody response to a T-cell-dependent antigen [127, 128]. Recently, this type of analysis has also been extended to peripheral blood B cells, leading to the identification of some degree of intraclonal variation in circulating clones compared to bone marrow clones. Based on this finding, the existence of a common precursor that is subject to somatic mutation has been postulated [129].
Structural Features of Amyloidogenic Light Chains
Mutations in immunoglobulin light chains can exert a destabilizing effect on their structure [130–133], thus increasing their propensity to undergo misfolding and aggregation [134–138]. In this regard, crystallographic studies have been instrumental in analysing the similarities between amyloidogenic and non-amyloidogenic light chains [139, 140] and in determining the effect of specific mutations on the tertiary structure of the protein [141].
Compared to non-amyloidogenic light chains, the light chains associated with disease display a higher number of non-conservative mutations in specific structural regions, including the complementary determining regions 1 and 3, and some of these mutations are associated with serum free light chain levels in AL patients [142].
Post-translational modifications are believed to play a role in light chain amyloidogenicity, [143] and these include glycosylation, cysteinylation, tryptophan oxidation and truncation [144–148]. In general, data supporting the role of post-translational modifications in fibrillogenesis are scanty. The only exception is represented by truncation, whose role in amyloidogenesis has been extensively investigated based on the observation that AL deposits are mainly composed of the amino-terminal variable region and part of the constant region [143]. However, full-length light chains are found in the proteome of amyloid-laden tissue [149], and occasionally the constant region is the principal component of amyloid deposits [150]. The identity of the proteolytic enzymes responsible for this process remains obscure and, similarly, it is unknown whether proteolytic cleavage occurs before or after the monomeric light chain has been incorporated into higher-order aggregates. Biochemical studies based on a single recombinant light chain highlight a crucial role of the constant region at early stages of fibril formation [151] but whether this is a peculiar characteristic of the protein examined or a general feature of all amyloidogenic light chains is still to be determined.
Recently, proteomic studies on tissues from AL patients have supported the notion that peptides of the constant region of the amyloidogenic light chain are common constituents of amyloid deposits [149, 152–154]. Also, these analyses have been instrumental in identifying the hitherto-underestimated occurrence of cases–designated light + heavy chain amyloidosis or AHL amyloidosis—in which both the light and heavy chains of a monoclonal protein contribute to the formation amyloid deposits [155–157].
Mechanisms of Toxicity
As reported above, the exact mechanisms of tissue damage and organ dysfunction caused by the process of amyloid formation and deposition are not fully understood. Nonetheless, knowledge gained in the context of other forms of amyloidoses [71, 73, 158], as well as the growing body of evidence from experimental and clinical observations in AL, have contributed in recent years to significantly deepening our understanding of the pathophysiology of this disease. Key players in this process appear to be the immunoglobulin light chain fibril precursors. Indeed, exposure to physiologic levels of cardiotropic amyloidogenic light chains, in the absence of amyloid fibrils, can cause diastolic dysfunction in isolated mouse hearts [74] or inhibit pumping in the nematode—pharynx, which is evolutionary related to the vertebrate heart [159]. This leads to oxidative stress and impairs cell function [75], eventually resulting in apoptosis through the non-canonical p38α MAPK pathway [76, 160]. Whether this cellular response is elicited only in specific cell types or is a general event triggered by exposure to light chain oligomers needs to be determined.
Complementary evidence that prefibrillar species, rather than fibrillar deposits, are the culprit for most of the toxicity in AL amyloidosis comes from substantiated clinical findings. In particular, hematologic response to chemotherapy was shown to translate into significant improvement of organ function well before the resolution of amyloid deposits [161, 162]. These earlier observations have been subsequently corroborated by the discovery that chemotherapy-induced reduction of immunoglobulin free light chains, and presumably also of oligomers thereof, parallel the decrease of biochemical markers of cardiac dysfunction, despite an unchanged degree of myocardial amyloid deposits at echocardiography [163].
The cascade of events necessary for light chain oligomerization and the exact site where this process occurs are still enigmatic. Based on in vitro observations, immunoglobulin light chains can be internalized by cells through endocytosis [164, 165], and the existence of a putative cellular receptor mediating this process has been advocated [166]. Nonetheless, whether cellular internalization is a prerequisite for light chain toxicity or, alternatively, oligomers are built in the extracellular milieu and exert their detrimental effect through a different mechanism, remains to be elucidated.
Organ Tropism in AL Amyloidosis
With the exception of localized AL, where immunoglobulin light chain amyloid fibrils accumulate in proximity to the amyloidogenic plasma cell clone, amyloid deposits are mainly found at distal sites, in target organs, including the kidney, heart, liver and peripheral nervous system. Remarkably, any organ—excluding the central nervous system—can be potentially affected by this process (Fig. 2.3).


Fig. 2.3
The clinical spectrum of systemic AL amyloidosis. Clinical manifestations of systemic AL amyloidosis based on a clinical series of 1339 patients followed at our Center. Percentages refer to frequency at presentation. BNP brain natriuretic peptide, NT-proBNP amino-terminal fragment of proBNP, cTn cardiac troponins, ALP alkaline phosphatase, ESRD end stage renal disease
Laws governing the tissue tropisms of amyloidogenic light chains have not yet been elucidated. It has been hypothesized that the primary structure of the light chain plays a central role in determining tissue tropism, and this thinking is supported by the strong, albeit not absolute, association with IGLV6-57 light chains and predominant, or exclusive, kidney involvement [123–125]. The peculiar tropism of IGLV6-57 light chains for the kidney might be explained by a receptor-mediated interaction with mesangial cells [166, 167]. Recently, an association between soft tissue and bone involvement with IGKV1 family [168], as well as an association between dominant cardiac involvement and the gene IGLV1-44 [169] or IGLV3 family [170], has been described. However, the germ-line gene alone is not sufficient to explain the phenomenon of tissue tropism of amyloidogenic light chains, as the pattern of amyloid deposition in individuals with the same germ-line gene origin can differ substantially [171]. Other factors, including somatic mutations and post-translational modifications, are likely to be involved [171].
Clinical Manifestations
From a clinical point of view, systemic AL amyloidosis is a truly protean condition [31, 172]. Indeed, depending on the number and types of organs involved, highly heterogeneous clinical manifestations can arise (Fig. 2.3).
A few manifestations, including conspicuous macroglossia, periorbital purpura and the shoulder pad sign (Fig. 2.3), can be regarded as almost pathognomonic (with few exceptions [173]) for systemic AL amyloidosis. They should, therefore, greatly favour a diagnosis of systemic amyloidosis and guide the physician towards a correct typing as AL [31, 172]. Nonetheless, these manifestations are rather uncommon, being present in no more than 15–20 % of cases. Involvement of soft tissues can also manifest as carpal tunnel syndrome due to amyloid deposition within the carpal canal [168]. This condition is often bilateral and can precede the clinical onset of other organ involvement by many years.
In the clinical series of 1339 patients with systemic AL amyloidosis followed at our Center (Table 2.1), the most frequently affected organs are kidneys and heart [174]. Renal involvement [175–178] results almost invariably in proteinuria, which can be prominent and can lead to severe hypoalbuminemia. More than 50 % of AL patients present with nephrotic syndrome at diagnosis. In contrast, renal insufficiency is infrequent at clinical onset, even though approximately 20 % of patients eventually develop terminal kidney failure and require dialysis. Progression of renal damage depends on residual organ function as well as on the severity of proteinuria [178, 179]. This can, however, be halted by effective therapy [178, 180], which underlines the paramount importance of an early diagnosis and timely treatment initiation. The ability to replace the organ function through dialysis explains why renal involvement in AL has a lesser impact on survival than cardiac involvement. Despite significant morbidities associated with dialysis, most AL patients with renal involvement eventually die because of amyloid-related cardiac dysfunction.
Table 2.1
Clinical presentation of AL amyloidosis
Organ or syndrome | Occurrencea | Overt clinical presentation | Early red flags |
---|---|---|---|
Heart | 70 % | • Heart failure • Arrhythmias • Restrictive cardiac wall thickening • Low electrocardiographic voltage • Late gadolinium enhancement at MRI | • NTproBNP > 332 ng/L (100 % sensitivity) • BNP > 73 ng/L (89 % sensitivity) |
Kidney | 70 % | • Nephrotic syndrome • Renal failure | • Proteinuria > 0.5 g/day (predominantly albumin) |
Liver | 22 % | • Hepatomegaly without scan defects | • Elevation of ALP or γGT in the absence of other causes |
PNS/ANS | 14 % | • Symmetric ascending peripheral neuropathy (small fiber, axonal) • Postural hypotension • Bladder and bowel dysfunction | • Neuropathic pain and loss of sensitivity to temperature • Onset of hypotension or resolution of hypertension |
Soft tissues | 13 % | • Purpura (periorbital) • Macroglossia • Claudication of the jaw • Muscular pseudohypertrophy • Articular deposits | • Carpal tunnel syndrome |
General symptoms | 74 % | • Malnutrition | • Unexplained fatigue • Weight loss |
At diagnosis, two-thirds of patients show echocardiographic signs of cardiac amyloidosis, consisting mainly of increased interventricular septum and posterior wall thicknesses, and often reduced internal ventricular chamber dimensions [181, 182]. Amyloid infiltrates within the myocardium may result in a characteristic granular sparkling appearance (Fig. 2.3). Global systolic function, as estimated by ejection fraction, is usually preserved at presentation, but tends to deteriorate with disease progression. Increased ventricular wall thicknesses are paradoxically accompanied by low voltage and a pseudo-infarction pattern with Q waves in precordial leads at ECG examination (Fig. 2.3). Recently, the usefulness of cardiac magnetic resonance [183] and left ventricular strain imaging [184–187], both for the diagnosis of AL cardiomyopathy and for prognostic assessments of AL patients, has been reported. Also, molecular imaging with amyloidotropic radiotracers is emerging as a useful diagnostic tool to investigate amyloid cardiomyopathy [188].
Clinically, amyloid cardiomyopathy manifests as right-sided heart failure. The presence and severity of cardiac involvement strongly influence the prognosis [189]. The severity of heart dysfunction can be quantified through the cardiac biomarkers N-terminal natriuretic peptide type B (NT-proBNP) and cardiac troponins (cTn) [190–193], which form the basis for a prognostic stratification [194–196] and can be used to monitor organ response to therapy [163, 192, 195]. Heart involvement is the leading cause of death in AL: 50 % of patients die due to chronic heart failure and 25 % die from sudden cardiac death due to fatal arrhythmias. Complex ventricular arrhythmias on 24-h ECG Holter monitoring, including couplets and non-sustained ventricular tachycardia, correlate with sudden death and are an independent prognostic factor [197]. Conduction disturbances are also frequently observed and negatively influence prognosis [198, 199].
Despite the great impact of cardiac involvement on prognosis, an effective treatment can significantly improve the survival of AL patients with cardiac amyloidosis, thus radically modifying the natural history of the disease [200].
Approximately, 22 % of AL patients present with liver involvement, resulting in hepatomegaly and/or elevated serum alkaline phosphatase levels [174, 201]. Jaundice is rare and, when present, often indicates a poor prognosis [202–204]. Rarely, hepatic amyloidosis can lead to spontaneous liver rupture, which is usually fatal [205, 206]. The spleen is generally affected by amyloid deposition, in some cases to a large extent, but splenic involvement is rarely of clinical relevance. When it does occur it leads to hyposplenism [207] and, anecdotally, to splenic rupture [208].
The involvement of the peripheral/autonomous nervous system is seen in about 14 % of patients [174] in the form of a predominantly sensitive, axonal, symmetrical and progressive neuropathy [209]. When peripheral neuropathy is the dominant syndrome, a differential diagnosis between AL and hereditary amyloidosis becomes mandatory. The presence of amyloid autonomic neuropathy manifests as postural hypotension, erectile dysfunction and gastrointestinal symptoms (constipation, diarrhoea or an alternation thereof). The latter symptoms can also be the consequence of amyloid deposition within the gastrointestinal tract, which is clinically evident in less than 10 % of cases. Other sites of amyloid deposition, including cutis, muscle, respiratory tract, genitourinary system and lymph nodes, are less commonly documented.
General symptoms, which are not explained by a specific pattern of organ involvement, are common and include fatigue—which is present in two-thirds of AL patients at presentation and can be rather severe—anorexia and dysgeusia. Unintentional weight loss is observed in more than 50 % of cases and can be masked by or underestimated because of concurrent liquid retention. Malnutrition, as assessed by low body mass index and low serum prealbumin level, is an important prognostic factor in AL [210–212].
Clinical Approach
In 2005, the International Society for Amyloidosis released consensus criteria for the definition of organ involvement and treatment response in AL amyloidosis, an unprecedented tool for physicians involved in the diagnosis and treatment of these patients [213]. These criteria have been subsequently updated and extended [178, 195, 214]. AL amyloidosis should be included in the differential diagnosis of: nondiabetic nephrotic syndrome; nonischemic cardiomyopathy with hypertrophic pattern on echocardiography; increased NT-proBNP in the absence of primary heart disease; hepatomegaly and/or increased alkaline phosphatase levels with no imaging abnormalities of the liver; peripheral and/or autonomic neuropathy; unexplained facial or neck purpura or macroglossia; association of monoclonal component with unexplained fatigue, weight loss, edema or paresthesia.
Diagnosis is based on the histological demonstration of amyloid deposits and determination of the amyloid type. Diagnosis of amyloid as AL in tissue samples necessitates a search for the plasma cell clone responsible for the production of amyloidogenic light chains, which is best achieved by a combination of clinical chemistry and hematologic investigations [215, 216]. However, once the coexistence of a monoclonal gammopathy and systemic amyloidosis has been ascertained, the possibility of a fortuitous combination of non-AL systemic amyloidosis (AA, wild-type ATTR or hereditary) and incidental, unrelated paraproteinemia should formally be taken into account [217–219]. This holds true especially in elderly subjects, due to the remarkable prevalence of monoclonal gammopathies of undetermined significance (MGUS) in this setting [220]. On the other hand, the rare association of systemic AL amyloidosis with the presence of a potentially amyloidogenic mutation has been reported [221, 222]. Since treatment is radically different from one form of systemic amyloidosis to the other, amyloid typing is mandatory in tissue deposits and requires a scrupulous clinical evaluation and appropriate techniques, including immunohistochemistry, immune-electron microscopy, mass-spectroscopy-based methods and genetic testing [105].
Organ dysfunction can be halted, amyloid deposits can be slowly reabsorbed and survival can be significantly extended if the production of amyloidogenic light chains is zeroed or substantially reduced [1]. Possibly, due to the low proliferative profile of the underlying amyloidogenic plasma cell clone, a durable hematologic response to therapy can be achieved and translate into organ response, with a survival of 10 years or more [223, 224]. Based on these observations, the current therapeutic approach to systemic AL amyloidosis aims at eradicating the underlying plasma cell dyscrasia with chemotherapy, using regimens which are mainly adopted from anti-multiple myeloma therapies (Fig. 2.4). However, alternative approaches have also been considered. The anthracycline 4′-iodo-4′-deoxy-doxorubicin was shown to bind to amyloid fibrils both in vitro and in vivo and to promote amyloid clearance [225] (Fig. 2.4), although its clinical efficacy in AL amyloidosis is limited [226, 227]. Other strategies are currently under investigation in preclinical or clinical settings [228], including selective downregulation of the offending immunoglobulin light chain via anti-sense oligonucleotides [229] or small interfering RNA molecules [230–232] or, indirectly, through upregulation of ER quality control mechanisms [233], passive immunotherapy with anti-amyloid antibodies [234, 235] and pharmacological depletion of SAP (Fig. 2.4) [44].


Fig. 2.4
Therapeutic options in AL amyloidosis. Similarly to anti-myeloma therapies, (1) chemotherapy and (2) proteasome inhibitors can be employed to eradicate amyloidogenic plasma cells. (3) Small molecules to increase ER quality control mechanisms can be applied to help attenuating secretion of amyloidogenic light chains. (4) Anti-sense oligonucleotides and (5) small interfering RNAs have been tested, in preclinical settings, to downregulate the production of the amyloidogenic light chains. (6) The palindromic compound (R)-1-[6-[(R)-2-carboxy-pyrrolidin-1-yl]-6-oxo-hexa-noyl]pyrrolidine-2 carboxylic acid (CPHPC) can be used to inhibit serum amyloid P component (SAP) binding to amyloid fibrils. The compound also crosslinks and dimerizes circulating SAP, favouring its hepatic clearance. This strategy can be combined with the use of (7) anti-SAP antibodies to eliminate visceral amyloid deposits. (8) Passive immunization with amyloid-reactive antibodies could promote the resolution of amyloid deposits. (9) The anthracycline 4′-iodo-4′-deoxy-doxorubicin (IDOX) interferes with amyloid fibril growth and promotes amyloid clearance
References
3.
Knowles TP, Vendruscolo M, Dobson CM. The amyloid state and its association with protein misfolding diseases. Nat Rev Mol Cell Biol. 2014;15:384–96.PubMed
4.
Fandrich M, Fletcher MA, Dobson CM. Amyloid fibrils from muscle myoglobin. Nature. 2001;410:165–6.PubMed
5.
Chiti F, Dobson CM. Protein misfolding, functional amyloid, and human disease. Annu Rev Biochem. 2006;75:333–66.PubMed
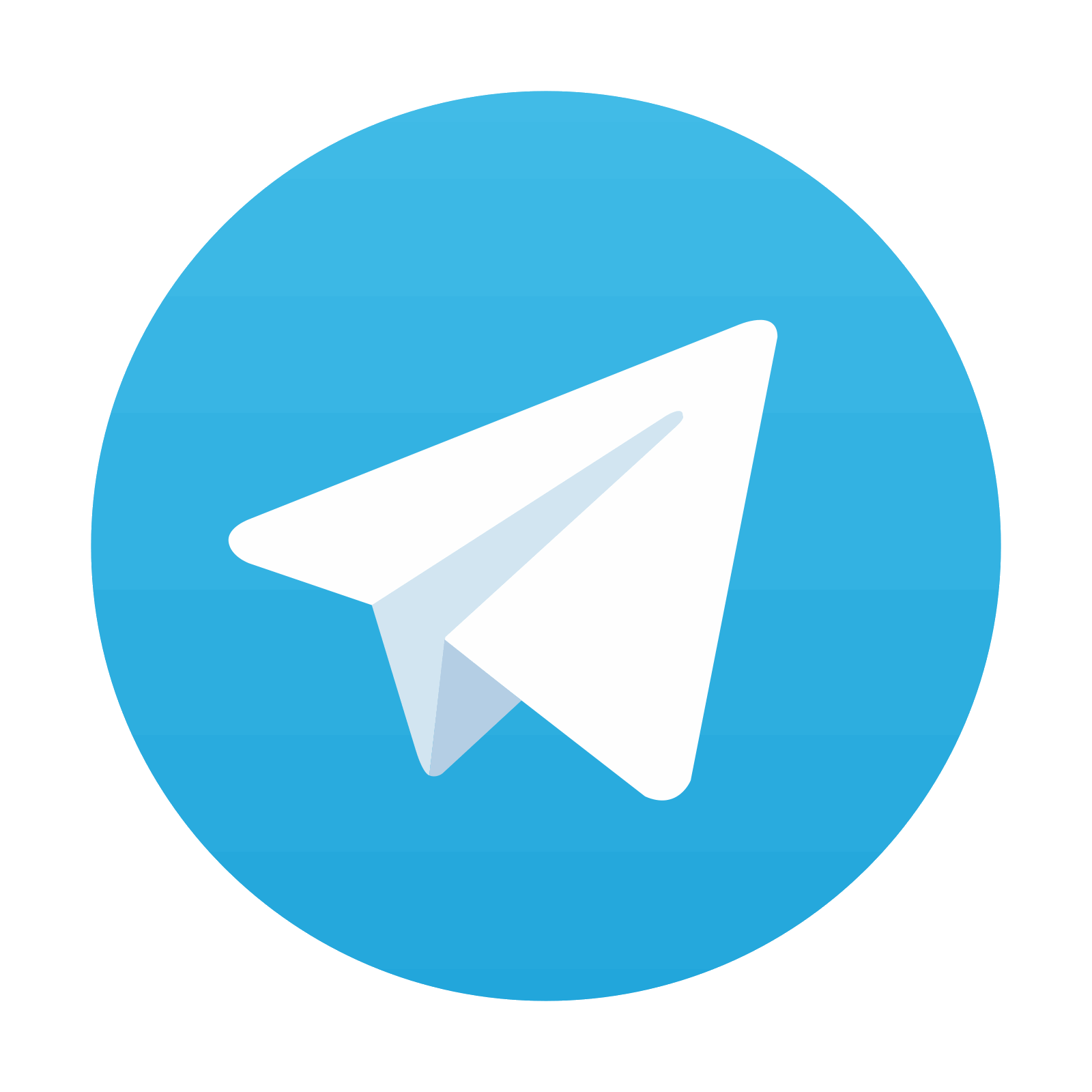
Stay updated, free articles. Join our Telegram channel
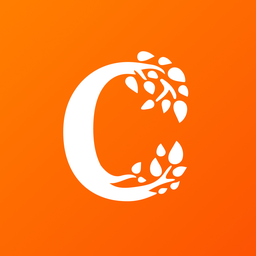
Full access? Get Clinical Tree
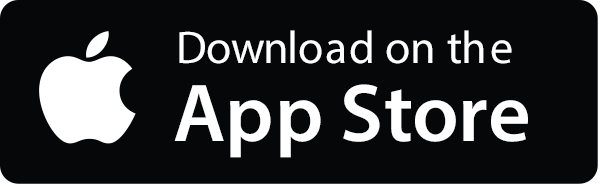
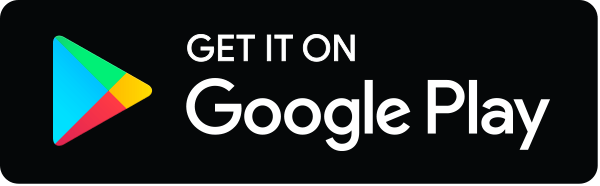