Table 10-1 AKIN and KDIGO Classification of AKI | ||||||||||||||||||
---|---|---|---|---|---|---|---|---|---|---|---|---|---|---|---|---|---|---|
|
causes of AKI were prerenal azotemia and urinary tract obstruction. Sepsis was the leading cause of AKI and more common than ischemic causes in the ICU (4, 17, 18, 19). The diseases may be primary renal or part of a systemic disease. The diseases of vessels and glomeruli will be dealt with in Chapter 15. This chapter therefore will focus primarily on the ischemic and nephrotoxic causes of AKI and acute interstitial nephritis (AIN).
Table 10-2 Conditions That Cause “Intrinsic” or Parenchymal AKI | ||||||||||||||||||||||||||||||||||||||||||||||||||||||||
---|---|---|---|---|---|---|---|---|---|---|---|---|---|---|---|---|---|---|---|---|---|---|---|---|---|---|---|---|---|---|---|---|---|---|---|---|---|---|---|---|---|---|---|---|---|---|---|---|---|---|---|---|---|---|---|---|
|
trigger caspase-independent necroptosis in five different cell types (41). These studies demonstrated that necroptosis is a major mechanism of proximal tubular cell death in AKI.
Table 10-3 Mediators/Mechanisms of Ischemic AKI | |||||||||||
---|---|---|---|---|---|---|---|---|---|---|---|
|
ischemic AKI had significantly lower BUN, serum creatinine, and ATN and apoptosis scores than the wild-type controls. The difference in protection against cisplatin-induced AKI compared with ischemic AKI in NLRP3(-/-) mice was not explained by the differences in proinflammatory cytokines IL-1β, IL-6, chemokine (C-X-C motif) ligand 1, or tumor necrosis factor-α (TNF-α). Thus the NLRP3 inflammasome is a mediator of ischemic AKI but not cisplatin-induced AKI (142).
Tg AKI versus wild-type AKI kidneys. This study demonstrates that protection against ischemic AKI in IL-18BP Tg mice is associated with less macrophage infiltration and less production of CXCL1 in the kidney.
![]() Figure 10-2 Calpains and caspases in proximal tubular necrosis. Hypoxic/ischemic proximal tubular necrosis results in activation of cysteine protease pathways involving calpains and both caspase-1 and caspase-3 (164). There is increased activity of calpain (113, 114, 115) and caspase-1 (153) in hypoxic proximal tubular injury. During ischemic AKI, there is early calpain activation associated with downregulation of calpastatin protein, decreased calpastatin activity, and activation of caspase-3 (163). Also, impaired IL-18 processing protects caspase-1-deficient mice from ischemic AKI (158). |
therefore called inducible NOS (iNOS) (170). iNOS expression results in sustained production of NO. Unlike cNOS, iNOS activity is believed to be insensitive to changes in intracellular Ca2+, since calmodulin is tightly bound to the molecule. Once synthesized, iNOS remains tonically activated, producing NO continuously for the life of the enzyme (171).
and/or adherence to the tubular basement membrane. Nidogen-1 (entactin), which acts as a bridge between the extracellular matrix molecules, laminin-1 and type IV collagen breakdown products, is produced as the result of partial degradation of tubular basement membrane by meprin A following renal tubular I/R injury (187).
![]() Figure 10-3 Proposed imbalance of NO production in ischemic/septic AKI. In ischemic AKI, increased NO derived from iNOS is damaging to proximal tubules (176, 177, 179). In ischemic AKI, renal endothelial damage results in decreased NO derived from eNOS (26). In endotoxemic AKI, increased iNOS activity decreases eNOS activity possibly via NO autoinhibition (387). The nonselective NOS inhibitor, L-NAME, worsens ischemic and endotoxemic AKI due to an overriding blocking effect on eNOS. |
prevented caspase-3 activation, and attenuated cell death in response to oxidative stress (233). In a rat model of hemorrhagic shock, administration of EPO before resuscitation reduced the increase in the activities of caspase-3, caspase-8, and caspase-9, and prevented renal dysfunction and liver injury (234). In a model of endotoxemia-induced AKI in mice, EPO significantly decreased renal superoxide dismutase and attenuated the renal dysfunction as assessed by insulin-GFR (235).
Table 10-4 Erythropoietin Protects against AKI | ||||||||||||||||||||||||||||||||||||||||||||||||||||||||||||||||||
---|---|---|---|---|---|---|---|---|---|---|---|---|---|---|---|---|---|---|---|---|---|---|---|---|---|---|---|---|---|---|---|---|---|---|---|---|---|---|---|---|---|---|---|---|---|---|---|---|---|---|---|---|---|---|---|---|---|---|---|---|---|---|---|---|---|---|
|
models (261). NGAL forms a complex with iron-binding siderophores and stops inappropriately liganded iron from producing damaging oxygen radicals (264).
mitochondrial fragmentation, while restoration of SIRT3 with 5-Aminoimidazole-4-carboxamide ribonucleotide (AICAR) and ALCAR improved cisplatin-induced mitochondrial dysfunction. This study suggests that SIRT3 improves mitochondrial dynamics in AKI (277).
its regulation. For instance, there may be a progressive loss of regional organ blood flow following I/R. There also may be an exaggerated constriction to neurohumoral agonists, failure to respond to physiologic and pharmacologic vasodilators, and paradoxical vasoconstrictor responses to changes in arterial pressure and blood flow following a period of transient organ ischemia and reperfusion. Evidence suggests that disordered vascular function subsequent to I/R injury may itself have a substantial impact on organ recovery, since normalization of blood flow influences the rate of parenchymal cell restoration.
Table 10-5 Factors That Modify Vascular Tone | ||||
---|---|---|---|---|
|
reactivity is strikingly different from that in the renal artery clamp AKI model. The difference likely is due to less severe ischemia and a slower rate of reperfusion. There is an exaggerated renal vasoconstrictor response to angiotensin II and endothelin-1 (ET-1) both in vivo and in arterioles isolated from these kidneys (296, 302). The response to endothelium-dependent vasodilators is reduced, but the constrictor response to L-NAME is actually increased (296). cNOS can be identified as at least as strongly reactive or more reactive than normal, as determined with cNOS monoclonal antibody in the resistance arterial vessels (303). While there is a dilator response to cyclic adenosine monophosphate-dependent PGI2 in the 48-hour postischemic renal vasculature, there is no increase in renal blood flow to the NO donor sodium nitroprusside. Taken together, these data indicate that at 48 hours after ischemia in NE AKI in the rat kidney, vascular cNOS activity is not diminished but rather is maximal such that it cannot be stimulated further by endothelium-dependent vasodilators. The available NO under basal conditions has fully activated VSMC-soluble cyclic guanosine monophosphate such that there is no additional response to an exogenous NO donor.
of variously sized, differently colored dextrans or proteins. Movement of these molecules out of the microvasculature and accumulation within the interstitial compartment are readily observed during AKI. The FVB-TIE2/GFP mouse, in which the endothelium is fluorescent, has been used to study morphologic changes in the renal microvascular endothelium during I/R injury in the kidney (313). Alterations in the cytoskeleton of renal microvascular endothelial cells correlated with a permeability defect in the renal microvasculature as identified using fluorescent dextrans and two-photon intravital imaging. This study demonstrates that renal vascular endothelial injury occurs in ischemic AKI and may play an important role in the pathophysiology of ischemic AKI.
an actual reduction in eNOS activity or to an actual spontaneous maximal NOS/NO activity that cannot be stimulated further by endothelium-dependent agents.
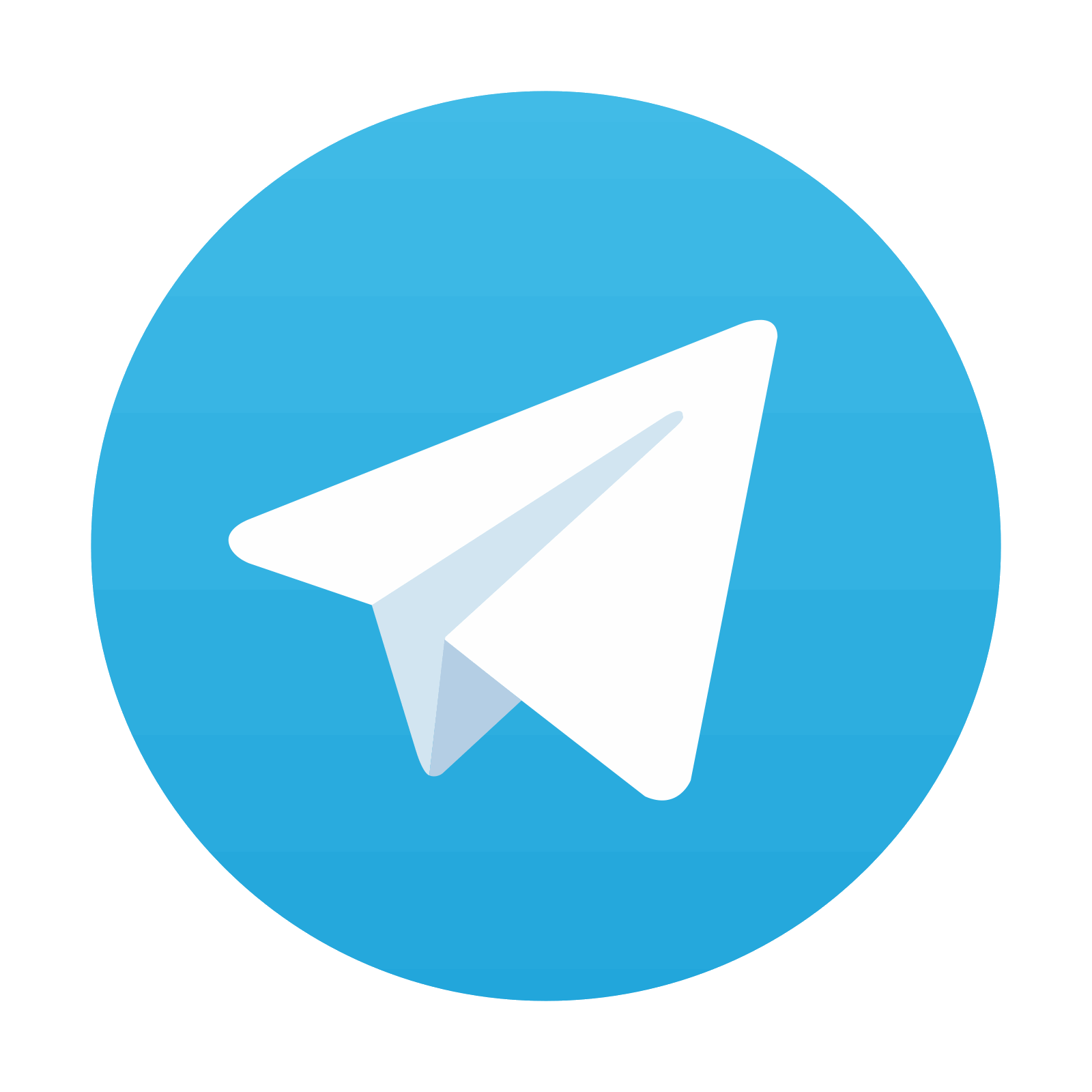
Stay updated, free articles. Join our Telegram channel
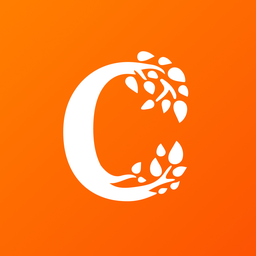
Full access? Get Clinical Tree
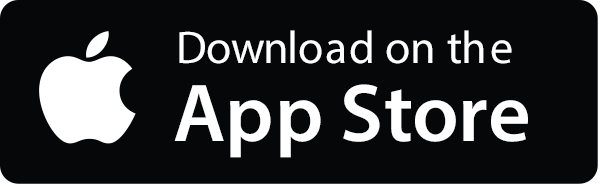
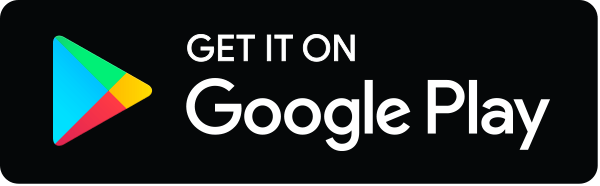
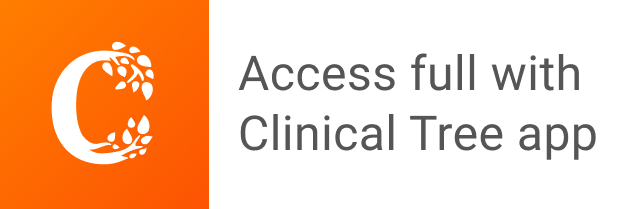