Acid-Base Disturbances
The importance of acid-base homeostasis cannot be overstated because of its importance in keeping hydrogen ion (H+) balance under control. Acid-base abnormalities are relatively common medically, and it is therefore essential for clinicians to be fully conversant with their interpretation.
Our cells release between 50 and 100 mmol of H+ into the extracellular fluid (ECF) daily. Despite this, the extracellular H+ concentration ([H+]) is maintained at about 40 nmol/L (pH 7.4).
The predominant sources of H+ are:
Anaerobic carbohydrate metabolism produces lactate, and anaerobic metabolism of fatty acids by β-hydroxylation and of ketogenic amino acids produces acetoacetate, which releases equimolar amounts of H+. Lactic acidosis or ketoacidosis can occur if the release of H+ by these reactions exceeds the compensatory capacity.
Release of H+ can occur during conversion of amino nitrogen to urea in the liver, or of the sulphydryl groups of some amino acids to sulphate.
Hydrogen ion balance is largely dependent upon the secretion of H+ from the body into the urine due to renal tubular action. Aerobic metabolism of the carbon skeletons of organic compounds converts carbon, hydrogen and oxygen into carbon dioxide and water. Carbon dioxide is an essential component of the extracellular buffering system. Thus the body is dependent upon healthy function of the kidneys and the lungs for normal acid-base homeostasis.
DEFINITIONS
Acids can dissociate to produce H+ (protons), which can be accepted by a base. An alkali dissociates to produce hydroxyl ions (OH−). Acidosis is commoner than alkalosis because metabolism tends to produce H+ rather than OH−.
A strong acid is almost completely dissociated in aqueous solution, and so produces many H+. For example, hydrochloric acid is a strong acid and is almost entirely dissociated in water to form H+ and chloride (Cl−). Weak acids dissociate less, although very small changes in [H+] may have important consequences.
Buffering is a process by which a strong acid (or base) is replaced by a weaker one, with a consequent reduction in the number of free H+, and therefore the change in pH, after addition of acid, is less than it would be in the absence of the buffer. For example:

The pH is a measure of H+ activity. It is log 10 of the reciprocal of [H+] in mol/L. The log 10 of a number is the power to which 10 must be raised to produce that number:

If [H+] is 10-7 (0.0000001) mol/L, then -log [H+] = 7. But:

Therefore pH = 7.
A change of 1 pH unit represents a 10-fold change in [H+]. Changes of this magnitude do not normally occur in tissues. However, in pathological conditions the blood pH can change by more than 0.3 of a unit; a decrease of pH by 0.3, from 7.4 to 7.1, represents a doubling of the [H+] from 40 to 80 nmol/L. Thus, the use of the pH notation makes a very significant change in [H+] appear deceptively small.
A blood pH of 7.0 indicates a severe acidosis. A blood pH of 7.7 similarly indicates a severe alkalosis. Urinary
pH is much more variable than that of blood, and [H+] can vary 1000-fold (a change of 3 pH units).
pH is much more variable than that of blood, and [H+] can vary 1000-fold (a change of 3 pH units).
The Henderson-Hasselbalch equation expresses the relation between pH and a buffer pair – that is, a weak acid and its conjugate base. The equation is valid for any buffer pair, the pH being dependent on the ratio of the concentration of base to acid. Note that pKa is the negative logarithm of the acid dissociation constant (Ka), and, the larger the value of pKa, the smaller the extent of acid dissociation:

In this equation the base is bicarbonate (HCO3−) and the acid is carbonic acid (H2CO3). It is not possible to measure the latter directly; however, it is in equilibrium with dissolved CO2, of which the partial pressure (PCO2) can be estimated. The concentration of H2CO3 is derived by multiplying this measured value by the solubility constant (S) for CO2. Thus:

If the PCO2 is expressed in kPa, S = 0.23, or in mmHg, S = 0.03.
The overall pKa of the bicarbonate system is 6.1. Therefore if PCO2 is in kPa:

Plasma [HCO3−] is controlled largely by the kidneys and PCO2 by the lungs. In acid-base disturbances due to respiratory problems the kidneys are essential for compensation and, conversely, in metabolic (non-respiratory) causes of acid-base imbalance the compensation is due mainly to changes in pulmonary function.
Despite considerable fluctuations in the rate of release of H+ into the ECF, the [H+], and therefore pH, is relatively tightly controlled in blood by the following mechanisms.
H+ can be incorporated into water:
The reaction is reversible, and H+ combines with HCO3− only if the reaction is driven to the right by the removal of CO2. By itself, this would cause HCO3− depletion.
Buffering of H+ is a temporary measure, as the H+ has not been excreted from the body. The production of the weak acid of the buffer pair causes only a small change in pH (see Henderson-Hasselbalch equation). If H+ are not completely neutralized or eliminated from the body, and if production continues, buffering power will eventually be so depleted that the pH will change significantly.
There are two main ways by which H+ can be lost from the body: through the kidneys or some of the intestine, mainly the stomach. This mechanism is coupled with the generation of HCO3−. In the kidney this is the method by which secretion of excess H+ ensures regeneration of buffering capacity.
ACID-BASE CONTROL SYSTEMS
Carbon dioxide and H+ are potentially toxic products of aerobic and anaerobic metabolism respectively. Most CO2 is lost through the lungs, but some is converted to HCO3−, thus contributing important extracellular buffering capacity; inactivating one toxic product provides a means of minimizing the effects of the other.
A buffer pair is most effective at maintaining a pH near its pKa, that is, when the ratio of the concentrations of base to acid is close to 1. However, the optimum pH of the ECF is about 7.4, and the pKa of the bicarbonate system is 6.1. Although this may seem to be disadvantageous, the bicarbonate system is the most important buffer in the body because:
it accounts for more than 60 per cent of the blood buffering capacity,
H+ secretion by the kidney depends on it,
it is necessary for efficient buffering by haemoglobin (Hb), which provides most of the rest of the blood buffering capacity.
The control of carbon dioxide by the lungs
The partial pressure of CO2 (PCO2) in plasma is normally about 5.3 kPa (40 mmHg) and depends on the balance between the rate of production by metabolism and the loss through the pulmonary alveoli. The sequence of events is as follows:
Inspired oxygen (O2) is carried from the lungs to the tissues by Hb.
The tissue cells use the O2 for aerobic metabolism; some of the carbon in organic compounds is oxidized to CO2.
CO2 diffuses along a concentration gradient from the cells into the ECF and is returned by the blood to the lungs, where it is eliminated in expired air.
The rate of respiration, and therefore the rate of CO2 elimination, is controlled by chemoreceptors in the respiratory centre in the medulla of the brainstem and by those in the carotid and aortic bodies. The receptors respond to changes in the [CO2] or [H+] of plasma or of the cerebrospinal fluid. If the PCO2 rises much above 5.3 kPa, or if the pH falls, the rate of respiration increases.
Normal lungs have a very large reserve capacity for elimination of CO2. Not only is there a plentiful supply of CO2, the denominator in the Henderson-Hasselbalch equation, but the normal respiratory centre and lungs can control its concentration within narrow limits by responding to changes in the [H+] and therefore compensate for changes in acid-base disturbances.
Bicarbonate buffer system
The control of bicarbonate by the kidneys and erythrocytes
The erythrocytes and renal tubular cells generate HCO3−, the buffer base in the bicarbonate system, from CO2. Under physiological conditions:
the erythrocyte mechanism makes fine adjustments to the plasma [HCO3−] in response to changes in PCO2 in the lungs and tissues,
the kidneys play the major role in maintaining the circulating [HCO3−] and in eliminating H+ from the body.
The carbonate dehydratase system
Bicarbonate is produced following the dissociation of carbonic acid formed from CO2 and H2O. This is catalysed by carbonate dehydratase (CD; carbonic anhydrase), which is present in high concentrations in erythrocytes and renal tubular cells:

Erythrocytes and renal tubular cells have high concentrations of CD, but also have means of removing one of the products, H+; thus both reactions continue to the right, and HCO3− is formed. One of the reactants (water) is freely available and one of the products (H+) is removed. Bicarbonate ion generation is therefore accelerated if the concentration of:
CO2 rises,
HCO3− falls,
H+ falls because it is either buffered by erythrocytes or excreted from the body by renal tubular cells.
Therefore, an increase in intracellular PCO2 or a decrease in intracellular [HCO3−] in the erythrocytes and renal tubular cells maintains the extracellular [HCO3−] by accelerating the production of HCO3−. This minimizes changes in the ratio of [HCO2−] to PCO2 and therefore changes in pH.
In the normal subject, at a plasma PCO2 of 5.3 kPa (a [CO2] of about 1.2 mmol/L), erythrocytes and renal tubular cells maintain the extracellular HCO3− at about 25 mmol/L. The extracellular ratio of [HCO3−] to [CO2] (both in mmol/L) is just over 20:1. It can be calculated from the Henderson-Hasselbalch equation, and with a pKa of 6.1 this represents a pH very near 7.4. An increase of intracellular PCO2, or a decrease in intracellular [HCO3−], accelerates HCO3− production and minimizes changes in the ratio and therefore in pH.
Bicarbonate generation by the erythrocytes (Fig. 4.1)
Haemoglobin is an important blood buffer. However, it only works effectively in cooperation with the bicarbonate system:

Erythrocytes produce little CO2 as they lack aerobic pathways. Plasma CO2 diffuses along a concentration gradient into erythrocytes, where CD catalyses its reaction with water to form carbonic acid (H2CO3), which then dissociates. Much of the H+ is buffered by Hb, and the HCO3− diffuses out into the extracellular fluid along a concentration gradient.
Electrochemical neutrality is maintained by diffusion of Cl− in the opposite direction into cells. This movement of ions is known as the ‘chloride shift’.
Under normal circumstances the higher PCO2 in the blood leaving tissues stimulates erythrocyte HCO3− production; consequently the arteriovenous difference in the ratio of [HCO3−] to [CO2], and therefore the pH, is kept relatively constant.
Extracellular and intracellular buffers other than HCO3− and Hb do not contribute significantly to blood buffering. They include:
phosphate, which has a plasma concentration of about 1 mmol/L, but a higher concentration in bone and inside cells where buffering capacity is of more importance,
proteins, which, because of their low concentrations in plasma, also have little blood-buffering capacity.
The kidneys
Carbonate dehydratase is also of central importance in the mechanisms involved in H+ secretion and in maintaining the HCO3− buffering capacity in the blood. Hydrogen ions are secreted from renal tubular cells into the lumina, where they are buffered by constituents of the glomerular filtrate. Unlike Hb in erythrocytes, urinary buffers are constantly being replenished by continuing glomerular filtration. For this reason, and because most of the excess H+ can only be eliminated from the body by the renal route, the kidneys are of major importance in compensating for chronic acidosis. Without them, the Hb buffering capacity would soon become saturated.
Two renal mechanisms control [HCO3−] in the ECF:
Bicarbonate reclamation (‘reabsorption’), the predominant mechanism in maintaining the steady state. The CO2 driving the CD mechanism in renal tubular cells is derived from filtered HCO3−. There is no net loss of H+ (Fig. 4.2).
Bicarbonate reclamation
Normal urine is nearly HCO3− free. An amount equivalent to that filtered by the glomeruli is returned to the body by the tubular cells. The luminal surfaces of renal tubular cells are impermeable to HCO3−. Thus, HCO3− can only be returned to the body if first converted to CO2 in the tubular lumina, and an equivalent amount of CO2 is converted to HCO3− within tubular cells. The mechanism depends on the
action of CD, both in the brush border on the luminal surfaces and within tubular cells, and on H+ secreted into the lumina in exchange for sodium (Na+). This occurs predominantly in the proximal tubules but also in the first part of the distal tubules:
action of CD, both in the brush border on the luminal surfaces and within tubular cells, and on H+ secreted into the lumina in exchange for sodium (Na+). This occurs predominantly in the proximal tubules but also in the first part of the distal tubules:
![]() Figure 4.2 Normal reabsorption of filtered bicarbonate from the renal tubules. CD, carbonate dehydratase. |
![]() Figure 4.3 Net generation of bicarbonate by renal tubular cells with excretion of hydrogen ions. B−, non-bicarbonate base; CD, carbonate dehydratase. |
Bicarbonate is filtered through the glomeruli at a plasma concentration of about 25 mmol/L.
Filtered HCO3− combines with H+, secreted by tubular cells, to form H2CO3.
The H2CO3 dissociates to form CO2 and water. In the proximal tubules this reaction is catalysed by CD in the brush border. In the distal tubules, where the pH is usually lower, H2CO3 probably dissociates spontaneously.
As the luminal PCO2 rises, CO2 diffuses into tubular cells along a concentration gradient, and as the intracellular concentration of CO2 rises, CD catalyses its combination with water to form H2CO3, which dissociates into H+ and HCO3−.
Hydrogen ions are secreted in the tubular lumina in exchange for Na+ and so the HCO3−-generating reactions start again from the second stage. As the intracellular concentration of HCO3− rises, HCO3− diffuses into the ECF accompanied by Na+, which has been reabsorbed in exchange for H+.
This self-perpetuating cycle reclaims buffering capacity that would otherwise have been lost from the body by glomerular filtration. The secreted H+ is derived from cellular water, and is incorporated into water in the lumina. Because there is no net change in H+ balance and no net gain of HCO3−, this mechanism cannot correct an acidosis but can maintain a steady state.
Bicarbonate generation
The mechanism in renal tubular cells for generating HCO3− is identical to that of HCO3− reabsorption, but there is net loss of H+ from the body as well as a net gain of HCO3−. Therefore this mechanism is well suited to correcting any type of acidosis.
Within tubular cells, CD may be stimulated by the following.
A rise in PCO2: in this case, the rise in [CO2] is the indirect result of a rise in the extracellular PCO2. Renal tubular cells, unlike erythrocytes with anaerobic pathways, constantly produce CO2 aerobically. This diffuses out of cells into the extracellular fluid along a concentration gradient. An increase in extracellular PCO2, by reducing the gradient, slows this diffusion and the intracellular PCO2 rises.
A fall of [HCO3−]: reduction of extracellular [HCO3−], by increasing the concentration gradient across renal tubular cell membranes, increases the loss of HCO3− from cells.
Normally almost all the filtered HCO3− is reabsorbed. Once the luminal fluid is HCO3− free, continued secretion of H+ and the intracellular generation of HCO3− depend on the presence of other filtered buffer bases (B−). In their absence, the luminal acidity would increase so much that further H+ secretion would be inhibited. These buffers, unlike HCO3−, do not form compounds capable of diffusing back into tubular cells, nor is H+ incorporated into water.
There is net loss of H+ in urine as HB. The HCO3− formed in the cell is derived from cellular CO2 and therefore represents a net gain in HCO3−. Whenever 1 mmol of H+ is secreted into the tubular lumen, 1 mmol of HCO3− passes into the ECF with Na+. The mechanism of HCO3− generation is very similar to that in erythrocytes. However, unlike red cells, renal tubular cells are exposed to a relatively constant PCO2. Bicarbonate generation, coupled with H+ secretion, becomes very important in acidosis, when it is stimulated by either a fall in extracellular [HCO3−] (metabolic acidosis) or a rise in extracellular PCO2 (respiratory acidosis).
Urinary buffers
Apart from HCO3−, the two other major urinary buffers are phosphate and ammonia (NH3); they are also involved in HCO3− generation.
Phosphate buffer pair
At pH 7.4, most of the phosphate in plasma, and also in the glomerular filtrate, is monohydrogen phosphate (HPO42−), which can accept H+ to become dihydrogen phosphate (H2PO4−). Bicarbonate can continue to be generated within tubular cells, with H+, and to be returned to the body after all that in the filtrate has been reabsorbed. Therefore it can help to replace that used in extracellular buffering. The pKa of this buffer pair is about 6.8:

Phosphate is normally the most important buffer in the urine because its pKa is relatively close to the pH of the glomerular filtrate and because the concentration of phosphate increases 20-fold, to nearly 25 mmol/L, as water is reabsorbed from the tubular lumen.
Even in a mild acidosis, more phosphate ions are released from bone than at normal pH; the need for increased urinary H+ secretion is linked with increased buffering capacity in the glomerular filtrate owing to the increase of phosphate. At a urinary pH below 5.5, most of the filtered phosphate is converted to dihydrogen phosphate. Therefore, at low pH, urinary phosphate cannot maintain the essential buffering of continued H+ secretion. The predominant urinary anion is Cl−, but, because hydrochloric acid is almost completely ionized in aqueous solution, it cannot act as a buffer.
The role of ammonia
As the urine becomes more acid, it can be shown to contain increasing amounts of ammonium ion (NH4 +). Urinary ammonia probably allows H+ secretion, and therefore HCO3− formation, to continue after other buffers have been depleted.
Ammonia, produced by hepatic deamination of amino acids, is rapidly incorporated into urea, with a net production of H+. However, as the systemic [H+] increases, there is some shift from urea to glutamine (GluCONH2) synthesis, with a slight fall in hepatic H+ production.
Glutamine is taken up by renal tubular cells, where it is hydrolysed by glutaminase to glutamate (GluCOO−) and NH4+:

Ammonia and NH4 + form a buffer pair with a pKa of about 9.8:

Because of the very high pKa, at pH 7.4 and below the equilibrium is overwhelmingly in favour of NH4+ production. Ammonia can diffuse out of the cell into the tubular lumen much more rapidly than NH4+. If the luminal fluid is acidic, NH3 will be retained within the lumen by avid combination with H+ derived from the CD mechanism. This allows H+, produced in the kidneys, to be excreted as ammonium chloride (NH4 +Cl−); thus, in severe acidosis, HCO3− formation can continue even when phosphate buffering power has been exhausted. There is a net gain of HCO3−. However, H+ as well as NH3 is released in renal tubular cells when NH4+ dissociates; this is maintained by passive diffusion of NH3 into the luminal fluid (Fig. 4.4).
On the face of it there seems no advantage in buffering one H+ secreted into the lumen if, at the same time, another is produced within the cell.
Explanations for the role of NH3 in the correction of acidosis include the following:
The fate of the GluCOO− produced at the same time as the NH4+. After further deamination to 2-oxoglutarate, it can be converted to glucose; gluconeogenesis uses an amount of H+ equivalent to that used in the generation of NH4+ produced from glutamine. Therefore, the H+ liberated into the cell may be incorporated into glucose.
A shift from urea synthesis to glutamine production in the liver with a fall in systemic H+ production in the presence of an acidosis. This is a minor factor.
The rate of gluconeogenesis and glutamine synthesis and glutaminase activity all increase in an acidosis.
![]() Figure 4.4 The role of ammonia in the generation of bicarbonate by renal tubular cells. CD, carbonate dehydratase. Modified with kind permission from Williams DL, Marks V (eds), Biochemistry in Clinical Practice. London: Heinemann Medical Books, 1983. © Elsevier. |
Bicarbonate formation in the gastrointestinal tract
Carbonate dehydratase also catalyses the formation of HCO3− in intestinal mucosal cells. The HCO3− may pass either into the ECF or into the intestinal lumen – a mechanism that can continue only if H+ is pumped in the opposite direction. Electrochemical neutrality is maintained by one of two mechanisms:
Na+ exchange for H+, by a mechanism that is the opposite of that in renal tubular cells,
passage of Cl− with H+.
Acid secretion by the stomach
The parietal cells of the stomach secrete H+ into the lumen together with Cl−. As H+Cl− enters the gastric lumen, HCO3− diffuses into the ECF, thus accounting for the post-prandial ‘alkaline tide’. In the healthy subject this is rapidly corrected by HCO3− secretion, mainly by the pancreas, as food passes down the intestinal tract. This mechanism explains the metabolic alkalosis that occurs in pyloric stenosis.
Sodium bicarbonate secretion by pancreatic and biliary cells
Sodium bicarbonate secretion by the pancreatic and biliary cells in response to stimulation by secretin accounts for the alkalinization of the duodenal fluid and occurs by the reverse process of NaHCO3 reabsorption in renal tubular cells. The pancreatic and biliary mechanisms are accelerated by the local rise in PCO2 that results when H+ is pumped into the ECF and reacts with the HCO3− generated by gastric parietal cells. This is analogous to the stimulation of renal HCO3− formation by the rise in luminal PCO2. Loss of large amounts of duodenal fluid may cause HCO3− depletion.
Bicarbonate secretion and chloride reabsorption by intestinal cells
As fluid passes down the intestinal tract, HCO3− enters and Cl− leaves the lumen by a reversal of the gastric mucosal mechanism. Therefore the gastric loss of Cl− and the gain of HCO3− are finally corrected. Preferential reabsorption of urinary Cl− by this mechanism after ureteric transplantation into the ileum, ileal loops or the colon explains the hyperchloraemic acidosis (normal anion-gap acidosis) associated with this operation (Fig. 4.5).
ACID-BASE DISORDERS
Disturbances of H+ homeostasis always involve the bicarbonate buffer pair. In respiratory disturbances abnormalities of CO2 are primary, whereas in so-called metabolic or non-respiratory disturbances [HCO3−] is affected early and changes in CO2 are secondary.
Acid blood pH is known as acidaemia, and alkaline blood pH is called alkaemia. Abnormal processes, either respiratory or metabolic, generate abnormal amounts of acid or base – acidosis or alkalosis respectively. The blood pH may or may not be abnormal because of the compensatory mechanisms.
Acidosis or alkalosis can cause a variety of clinical symptoms including arrhythmias, weakness, confusion
and gastrointestinal problems. It is noteworthy that various enzymes have optimal pH values and acid-base disturbance can perturb their function.
and gastrointestinal problems. It is noteworthy that various enzymes have optimal pH values and acid-base disturbance can perturb their function.
CASE 1
A 7-year-old boy was admitted unconscious to a casualty department. On examination he was found to be hyperventilating. He had inadvertently consumed ethylene glycol antifreeze, which he had found in his parents’ garage stored in a lemonade bottle. Blood results were as follows:
Plasma
Sodium 134 mmol/L (135-145)
Potassium 6.0 mmol/L (3.5-5.0)
Bicarbonate 10 mmol/L (24-32)
Chloride 93 mmol/L (95-105)
Glucose 5.3 mmol/L (3.5-6.0)
Arterial blood gases
pH 7.2 (7.35-7.45)
PaCO2 3.18 kPa (4.6-6.0)
PaO2 13.1 kPa (9.3-13.3)
DISCUSSION
The results show a high anion gap, normally about 15-20 mmol/L, but in this case 37 mmol/L, with metabolic acidosis, i.e. (134 + 6) – (10 + 93). Hyperkalaemia is present due to the movement of intracellular K+ out of cells because of the acidosis. The compensatory mechanism of hyperventilation ‘blows off’ volatile acid in the form of CO2, hence the low PCO2.
Acidosis
Acidosis occurs if there is a fall in the ratio of [HCO3−] to PCO2 in the ECF. It may be due to:
metabolic (non-respiratory) acidosis, in which the primary abnormality in the bicarbonate buffer system is a reduction in [HCO3−],
respiratory acidosis, in which the primary abnormality in the bicarbonate buffer system is a rise in PCO2.
Metabolic acidosis
The primary disorder in the bicarbonate buffer system in a metabolic acidosis is a reduction in [HCO3−], resulting in a fall in blood pH. The reduction in the HCO3− may be due to:
its use in buffering H+ more rapidly than it can be generated by normal homeostatic mechanisms,
loss in the urine or gastrointestinal tract more rapidly than it can be generated by normal homeostatic mechanisms,
impaired production.
The causes of a metabolic acidosis are shown in Box 4.1 and can be divided into those with a high and those with a normal plasma anion gap.
Concept of plasma anion gap
One negative charge balances one positive charge, and some substances are multivalent, having more than one charge per mole. If the molecular weight is divided by the valency, the charges on each resulting equivalent will be the same as those on an equivalent of any other chemical. Most of the ions in the following discussion are monovalent, and hence the number of millimoles is numerically the same as that of milliequivalents (mEq). However, when calculating ion balance, the latter notation should strictly be used, that is, concentration of charges.
Sodium and potassium (K+) provide more than 90 per cent of plasma cation concentration in the healthy subject; the balance includes low concentrations of calcium (Ca2+) and magnesium (Mg2+), which vary only by small amounts.
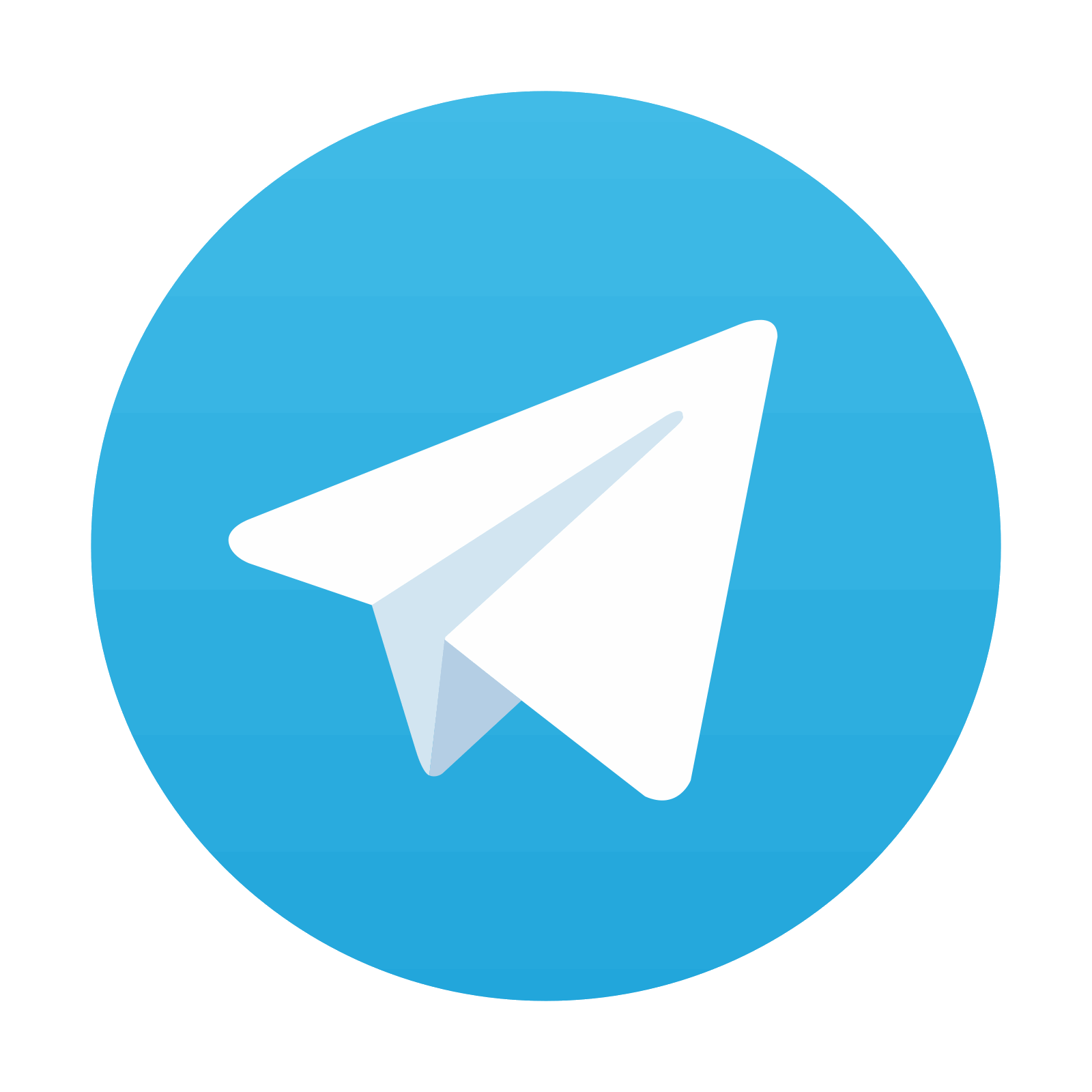
Stay updated, free articles. Join our Telegram channel
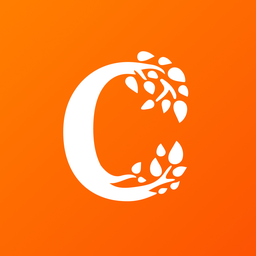
Full access? Get Clinical Tree
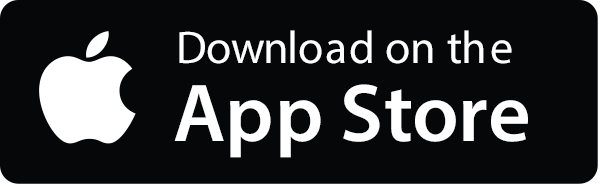
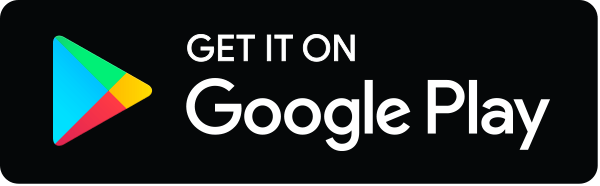
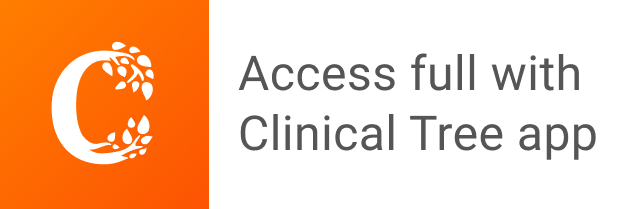