Fig. 4.1
Body size trajectories in the development of cardio-metabolic health
Clearly, the three trajectories in Fig. 4.1 are conceptual. While we split this section into life course stages for convenient handling of information, it is important to remember that each measure (e.g., weight and abdominal circumference) has its own trajectory that may follow a complex pattern of age-related change, demonstrate complex associations with cardio-metabolic health, and vary greatly between cohorts and across time, place, and population sub-groups. Further, traits in one trajectory might interact with traits in another trajectory to influence cardio-metabolic health. Considering birth weight and adulthood waist circumference, for example, risk for type two diabetes is greatest for individuals born light who went on to develop large waist lines (Tian et al. 2006).
Determinants and Mechanisms of Deleterious Trajectories
An association between body size at one age and cardio-metabolic health at a second age does not mean that the former causes the later. This section seeks to explain how and why body size across the life course may be associated with cardio-metabolic health.
Environmental Exposures
Foetal growth is ultimately restricted by uterine capacity. Babies may be born small because they are constrained in this way or because they lacked the nutrients in utero necessary for optimum growth (Harding 2001). Pregnant women exposed to famine give birth to small babies who go onto develop glucose intolerance and obesity in adulthood, for example (Ravelli et al. 1976, 1998). The proportion of protein and carbohydrate in a women’s diet may be a key factor that affects the foetus, with the combination of high carbohydrate and low protein intake being particularly deleterious (Campbell et al. 1996; Godfrey et al. 1996). In addition to maternal intake per se, clinical exposures such as maternal hypertension leading to reduced uterine blood flow can severely affect the supply line of nutrients from the mother to the foetus (Harding 2001).
Other well-known determinants of size at birth include sex, gestational age, ethnic origin, parity, maternal and paternal size, gestational weight gain, general morbidity and episodic illness, malaria, cigarette smoking, and alcohol consumption (Kramer 1987). Maternal gestational diabetes is associated with higher birth weight and increased risk of subsequent obesity (Lawlor et al. 2011), but Gillman et al. (2003) have questioned the causal role of altered maternal-foetal glucose metabolism because adjusting the gestational diabetes-childhood obesity association for birth weight only marginally attenuated the estimate in their study. This might, however, be expected given that even the most exposed foetuses are limited to how much weight they can gain in utero; the greatest anatomical response to altered glucose metabolism will inevitably occur after birth, when there is no upper limit on weight gain. Indeed, the most deleterious profile for childhood obesity comprises rapid infant weight gain in addition to pre-pregnancy obesity and macroscomia (Weng et al. 2012).
Determinants of childhood obesity include maternal smoking, no or short duration of breastfeeding, obesity in infancy, short sleep duration, television viewing, low daily physical activity, and consumption of sugar-sweetened beverages (Monasta et al. 2010). Many of these risk factors continue to operate in adolescence (Morandi et al. 2012), at ages when there is increasing independence from the family and the establishment of more individual as compared to familial risk factors. Most research has focused on shifts in diet and physical activity as the key drivers of the obesity epidemic, particularly during adulthood (Swinburn et al. 2011; McAllister et al. 2009). There is, however increasing evidence for multiple other factors including microorganisms and epigenetics early in life, sleep debt, and endocrine disruptors (McAllister et al. 2009).
Naturally, the exposures responsible for obesity are very different to those for malnutrition. Evidence from low to middle income countries shows that the key determinants of stunting, wasting, and underweight include growth restriction in utero, poor condition of the mother, poverty, chronic dietary insufficiency, marked seasonality, poor levels of sanitation, and infection (Martorell and Young 2012; Frongillo et al. 1997). As these countries transition, it has been argued that the people are likely to face both sets of exposures and a dual burden of stunting and overweight (Varela-Silva et al. 2012). Others have, however, shown that the co-existence of stunting and overweight, at least at the family level, is a statistical artefact with a prevalence that matches what one would expect based on the separate stunting and overweight rates (Dieffenbach and Stein 2012).
Biological Pathways
Biological pathways are ultimately responsible for the links between environmental exposures, body size, and cardio-metabolic health. The first main type of pathway involves anatomical changes. Anatomical formation of the kidneys occurs exclusively in utero, and nutritional constraint of a foetus can permanently reduce the number of nephrons that are laid down, for example (Barker et al. 2006). Although physiological capability of the kidney develops over the entire life course, small kidney size in prenatally undernourished individuals increases risk for hypertension and renal failure in adulthood (Lampl et al. 2002; Luyckx and Brenner 2005). Similarly, a larger number of adipocytes in response to nutritional excess and a sedentary lifestyle in adulthood can increase CHD risk because these cells secrete inflammatory proteins that speed up the atherosclerotic process (Berg and Scherer 2005).
The second main type of pathway involves the physiological setting or alteration of hormonal and metabolic axes. For example, the foetus responds to reduced nutritional supply by reducing plasma concentrations of hormones, such as insulin and insulin-like growth factor, which in turn limits the transportation of glucose to the muscles and impairs lean tissue growth (Phillips 1996). This adaptation occurs so that glucose is readily available in the bloodstream to maintain growth of high priority organs, such as the brain (Gluckman 1995). The tendency to maintain high blood glucose levels can, however, lead to a progressive decline in glycaemic control and type two diabetes (Phillips 1996). As a different example, dysregulation of the hypothalamic-pituitary-adrenal axis in response to chronic stress in adulthood might lead through the effects of cortisol to central obesity and cardio-metabolic disease (Rosmond and Bjorntorp 2000).
One increasingly well-studied set of mechanisms focuses on the way in which genes governing body size impact on cardio-metabolic health. We know that environmental exposures can modify the expression of genes. A recent meta-analysis reported that the odds of obesity associated with the risk allele in the FTO gene was attenuated in active adults compared to inactive adults by 27 %, for example (Kilpelainen et al. 2011). Chemical modifications occur that alter gene expression in a specific tissue or organ without changing the nucleotide sequence of the DNA (e.g., methylation and histone modification) (Holliday 1994). While an environmental exposure may influence body size in the short term, these “epigenetic” changes can have long lasting effects on disease risk. Maternal protein restriction might lead to smaller offspring and reduce methylation (and therefore enhance expression) of the angiotensinogen receptor gene in the offspring adrenal gland, thereby leading to high blood pressure, for example (Bogdarina et al. 2007; Woodall et al. 1996). Such epigenetic changes can occur from conception onward but, like the other anatomical and physiological pathways, are most sensitive to the environment during specific stages of the life course.
Genes are also being used increasingly in Mendelian randomisation studies, where a genetic variant or set of variants are used as an instrumental variable for some phenotype or exposure, to establish whether or not that phenotype has a causal effect on some outcome (Lawlor et al. 2008). This technique has recently been used, for example, to demonstrate that BMI does have causal effects on various cardio-metabolic traits, including blood pressure and fasting glucose and cholesterol (Holmes et al. 2014). These studies work, subject to certain assumptions, on the premise that alleles are transmitted from parents to offspring randomly at gamete formation, such that the genotype or instrumental variable relationship with the outcome is not confounded by environmental factors or prone to reverse causation (Lawlor et al. 2008).
Critical Periods and Transitions
A critical period refers to an age window in which intrinsic changes in body structure and function are occurring rapidly and may be most easily programmed in a favourable or unfavourable direction (Scott 1986). The focus in the natural sciences was originally on the requirement of specific environmental stimuli to elicit the normal development and functioning of some body part or system (Cameron and Demerath 2002). In contrast, epidemiology focuses on the environmental exposures that result in anatomical and physiological adaptations that have long-term implications for cardio-metabolic health (Kuh et al. 2003). Perhaps the most often used example of a critical period is the teratogenic effect of maternal exposure to thalidomide in the first trimester of pregnancy on limb development in the foetus when the limbs are most rapidly developing, while exposure to thalidomide after birth is harmless (Newman 1986). In this instance, the critical period is truly critical. In other examples, however, the exposure association (with the outcome) is not constrained to such a narrow age window, but may be present across part or whole of the life course. The strongest associations are observed at ages when the individual is most sensitive to the environment, thus these are called sensitive periods of development.
Research has now identified potentially sensitive periods spanning the entire life course. Early childhood and adolescence in particular are emerging as important periods for the establishment of obesity and programming of cardio-metabolic risk. It has recently been shown in the MRC National Survey of Health and Development that socioeconomic disadvantage in early childhood, more so than at any other age, is associated with cardio-metabolic risk factors at age 53 years, for example (Murray et al. 2011). In the same cohort, exposure to obesity emerges during adolescence as being associated with greater carotid-intima media thickness at 60–64 years of age in men (Johnson et al. 2014). The associations reported in both of these papers were independent of birth weight and appeared to be mediated by higher adulthood BMI in those individuals who were disadvantaged in early childhood or obese in adolescence.
Adolescence is often viewed as critical from physical and behavioural perspectives, but individuals experience this stage in the life course at different ages and progress through secondary sexual characteristic maturation at different paces (Ellison and Reiches 2012). A critical or sensitive period may be thought of as a point of transition from one state to another. During puberty, for example, the adolescent transitions from an immature state to a mature state. Various markers of the pace of pubertal development have been reported to be associated with various cardio-metabolic health outcomes in both sexes (see section “Adolescence”). Interestingly, an early age at menopause, which marks the cessation of a women’s reproductive ability, is also associated with increased risk for cardio-metabolic disease (Ebong et al. 2014). Lifelong environmental conditions may influence the timing of menopause (Lawlor et al. 2003), but it is unknown whether or not the biological changes that occur during menopause and impact on subsequent health are particularly sensitive to concurrent exposures. Similarly, behavioral transitions throughout the life course can lead to increased disease risk, but these do not necessarily occur at ages when biological changes are occurring rapidly and are most sensitive to the environment. Transition into a romantic relationship or marriage can cause behavioral changes that lead to the development of obesity (The and Gordon-Larsen 2009; Gordon-Larsen et al. 2004), but there is nothing biologically critical about the exact age when this occurs, for example. The same might be true for starting university (Gropper et al. 2012), leaving the army (Littman et al. 2013), and retiring (Morris et al. 1992). The timing of rapid change in a known biological structure/function is critical for the experience of specific environmental stimuli to cause permanent alterations and predict long-term outcomes (Cameron and Demerath 2002). While a critical or sensitive period may be thought of as a transition, a transition does not automatically meet the requirements to be a critical of sensitive period.
Transgenerational Transmission
Recent research in human and animal models suggests that biological adaptations to environmental exposures during critical or sensitive periods of development may be transmitted to subsequent generations (Benyshek 2013), such that a trait like insulin resistance in the exposed generation might be passed to successive unexposed generations in diminishing order of magnitude (Benyshek et al. 2006). This transgenerational transmission goes beyond something that might be explained by genetic heritability or by family members of different generations experiencing similar lifelong environments. Maternal exposure to famine, for example, has been shown to be associated with increased risk of giving birth to small offspring with dysregulated lipid profiles (Lumey et al. 2009), who themselves go onto have relatively small offspring with large amounts of adiposity and high risk for cardio-metabolic disease (Painter et al. 2008). In this scenario, direct and simultaneous exposure of three generations (i.e., the pregnant mother, her fetus, and the fetuses’ primordial germ cells) might be responsible for the increased disease risk seen in each generation. Alternatively, and if more than three generations are affected, research is revealing how inheritance of epigenetic modifications to the genome may be responsible (Hackett et al. 2013).
Public Health Relevance and Conclusion
The role of life course research in public health debates, such as whether money should be spent on the primordial prevention of cardio-metabolic diseases in high risk strata of society or whether it should be used to provide effective treatment in the smaller number of people who actually develop a disease, is to provide clear empirical evidence of how and why a disease develops. Only then is it possible to understand what physical and social exposures should be targeted and at what ages. The conclusion of Barker et al. (1989) that “promotion of postnatal growth may be especially important in boys who weigh below 7.5 lb (3.4 kg) at birth” today may appear reckless. But this is only because a whole body of life course epidemiology literature has since been published showing that, at some ages and in some populations, rapid infant weight gain leads to the deleterious development of excess adiposity and may not incur protective long-term gains in height and fat-free mass (Bann et al. 2014; Druet et al. 2012; Kerkhof and Hokken-Koelega 2012; Wells et al. 2012). We may still not be at a situation where we should attempt to increase growth rates in infants who are born small, but we are developing a clearer picture of how, when, and in what populations this might be best achieved without adversely impacting on future health (Ong and Loos 2006).
Knowledge of the life course processes that lead to poor cardio-metabolic health and disease is relevant to many public health discussions and policies, not just those relating to infant growth and obesity. The life course perspective has become a central part of the World Health Organisation’s programme on non-communicable disease prevention and health promotion (World Health Organisation Dept of Noncommunicable Disease Prevention and Health Promotion 2001). Particularly in the UK, the importance of a life course approach is increasingly gaining recognition, with recent reports on mental wellbeing and reproductive health having all championed the approach (Foresight. 2008; Scientific Advisory Committee 2011). The Marmot (2010) review on reducing health inequalities in the UK included statements, such as “giving every child the best start in life” and “enabling all children, young people, and adults to maximise their capabilities and have controls over their lives”. The challenge life course epidemiologists now face is to fine-tune their studies to provide policy makers with the best, actionable information.
The literature on the relationships of body size with cardio-metabolic health is immense. Associations of body size with cardio-metabolic health can be explained in terms of anatomical and/or physiological changes in response to environmental conditions during critical or sensitive periods of development. These adaptations can persist across subsequent generations through epigenetic inheritance, thereby adding another layer of complexity to life course epidemiology. The integration of biological and social research is clearly important, as is the understanding of the processes and pathways operating across the life course. Taking this more holistic approach and understanding the life course trajectories of body size and cardio-metabolic health will lead to a more complete understanding of cardio-metabolic disease processes and how to stop disease processes progressing faster in some people than others.
Open Access This chapter is distributed under the terms of the Creative Commons Attribution Noncommercial License, which permits any noncommercial use, distribution, and reproduction in any medium, provided the original author(s) and source are credited.
References
Adair, L. S., Fall, C. H., Osmond, C., Stein, A. D., Martorell, R., Ramirez-Zea, M., et al. (2013). Associations of linear growth and relative weight gain during early life with adult health and human capital in countries of low and middle income: findings from five birth cohort studies. Lancet, 382(9891), 525–534.PubMedCentralPubMed
Bajekal, M., Scholes, S., O’Flaherty, M., Raine, R., Norman, P., & Capewell, S. (2013). Unequal trends in coronary heart disease mortality by socioeconomic circumstances, England 1982-2006: An analytical study. PloS One, 8(3), e59608.PubMedCentralPubMed
Bann, D., Wills, A., Cooper, R., Hardy, R., Aihie Sayer, A., Adams, J., et al. (2014). Birth weight and growth from infancy to late adolescence in relation to fat and lean mass in early old age: Findings from the MRC National Survey of Health and Development. International Journal of Obesity, 38(1), 69–75.PubMedCentralPubMed
Barker, D. J., & Osmond, C. (1986). Infant mortality, childhood nutrition, and ischaemic heart disease in England and Wales. Lancet, 1(8489), 1077–1081.PubMed
Barker, D. J., Winter, P. D., Osmond, C., Margetts, B., & Simmonds, S. J. (1989). Weight in infancy and death from ischaemic heart disease. Lancet, 2(8663), 577–580.PubMed
Barker, D. J., Bull, A. R., Osmond, C., & Simmonds, S. J. (1990). Fetal and placental size and risk of hypertension in adult life. British Medical Journal, 301(6746), 259–262.PubMedCentralPubMed
Barker, D. J., Godfrey, K. M., Fall, C., Osmond, C., Winter, P. D., & Shaheen, S. O. (1991). Relation of birth weight and childhood respiratory infection to adult lung function and death from chronic obstructive airways disease. British Medical Journal, 303(6804), 671–675.PubMedCentralPubMed
Barker, D. J., Godfrey, K. M., Osmond, C., & Bull, A. (1992). The relation of fetal length, ponderal index and head circumference to blood pressure and the risk of hypertension in adult life. Paediatric and Perinatal Epidemiology, 6(1), 35–44.PubMed
Barker, D. J., Osmond, C., Simmonds, S. J., & Wield, G. A. (1993). The relation of small head circumference and thinness at birth to death from cardiovascular disease in adult life. British Medical Journal, 306(6875), 422–426.PubMedCentralPubMed
Barker, D. J., Osmond, C., Forsen, T. J., Kajantie, E., & Eriksson, J. G. (2005). Trajectories of growth among children who have coronary events as adults. New England Journal of Medicine, 353(17), 1802–1809.PubMed
Barker, D. J., Bagby, S. P., & Hanson, M. A. (2006). Mechanisms of disease: In utero programming in the pathogenesis of hypertension. Nature Clinical Practice Nephrology, 2(12), 700–707.PubMed
Barker, D. J., Osmond, C., Kajantie, E., & Eriksson, J. G. (2009). Growth and chronic disease: Findings in the Helsinki Birth Cohort. Annals of Human Biology, 36(5), 445–458.PubMed
Beaglehole, R. (1999). International trends in coronary heart disease mortality and incidence rates. Journal of Cardiovascular Risk, 6(2), 63–68.PubMed
Belfort, M. B., & Gillman, M. W. (2013). Healthy infant growth: What are the trade-offs in the developed world? Nestle Nutrition Institute Workshop Series, 71, 171–184.PubMed
Ben-Shlomo, Y., & Kuh, D. (2002). A life course approach to chronic disease epidemiology: Conceptual models, empirical challenges and interdisciplinary perspectives. International Journal of Epidemiology, 31(2), 285–293.PubMed
Benyshek, D. C. (2013). The “early life” origins of obesity-related health disorders: New discoveries regarding the intergenerational transmission of developmentally programmed traits in the global cardiometabolic health crisis. American Journal of Physical Anthropology, 152(Suppl 57), 79–93.PubMed
Benyshek, D. C., Johnston, C. S., & Martin, J. F. (2006). Glucose metabolism is altered in the adequately-nourished grand-offspring (F3 generation) of rats malnourished during gestation and perinatal life. Diabetologia, 49(5), 1117–1119.PubMed
Berg, A. H., & Scherer, P. E. (2005). Adipose tissue, inflammation, and cardiovascular disease. Circulation Research, 96(9), 939–949.PubMed
Bogdarina, I., Welham, S., King, P. J., Burns, S. P., & Clark, A. J. (2007). Epigenetic modification of the renin-angiotensin system in the fetal programming of hypertension. Circulation Research, 100(4), 520–526.PubMedCentralPubMed
Cameron, N. (2007). Growth patterns in adverse environments. American Journal of Human Biology, 19(5), 615–621.PubMed
Cameron, N., & Demerath, E. W. (2002). Critical periods in human growth and their relationship to diseases of aging. American Journal of Physical Anthropology, 119(Suppl 35), 159–184.
Campbell, D. M., Hall, M. H., Barker, D. J., Cross, J., Shiell, A. W., & Godfrey, K. M. (1996). Diet in pregnancy and the offspring’s blood pressure 40 years later. British Journal of Obstetrics and Gynaecology, 103(3), 273–280.PubMed
Choi, J., Joseph, L., & Pilote, L. (2013). Obesity and C-reactive protein in various populations: A systematic review and meta-analysis. Obesity Reviews, 14(3), 232–244.PubMed
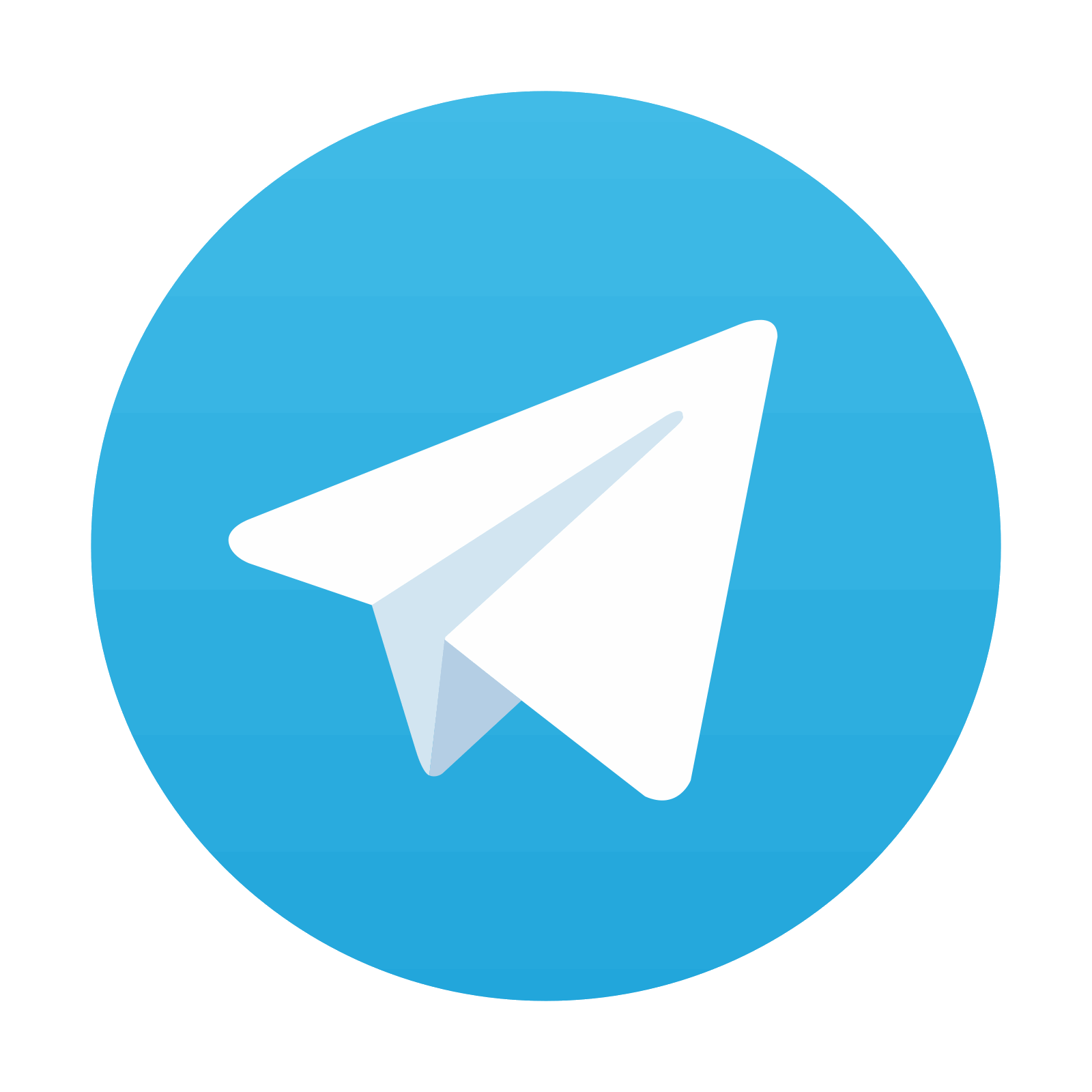
Stay updated, free articles. Join our Telegram channel
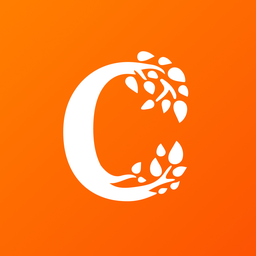
Full access? Get Clinical Tree
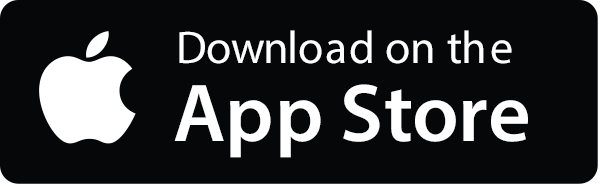
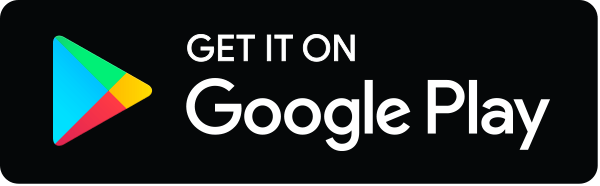