Bio-Inspired Integration of Natural Materials
3B’s Research Group—Biomaterials, Biodegradables, and Biomimetics, University of Minho, Headquarters of the European Institute of Excellence on Tissue Engineering and Regenerative Medicine, Guimarães, Portugal; ICVS/3B’s— PT Government Associate Laboratory, Braga/Guimarães, Portugal
8.1 Introduction
Natural extracellular matrices (ECMs) have been isolated and extracted from various tissues, such as small intestine submucosa, skin (from cadavers), pancreas, and breast [1]. Although these purified ECMs certainly have useful applications, their use is limited in scope, owing to the need for well-defined microenvironments in tissue regeneration and stem cell transplantation, in which animal by-products and contaminants must be limited. Moreover, it has been demonstrated that, besides the spatial framework, tissue-specific ECM cues are essential to regenerate an organ or tissue [2]. It is well established that ECM is dynamic and has an instructive role in building a tissue and in its regeneration after trauma or disease [2].
Tissue engineering (TE) has been recognized as a promising alternative to the use of autografts or allografts or even xenografts for tissue reconstruction and regeneration. Tissue engineering and regenerative medicine (TERM) aims at the development of biological substitutes that restore, maintain, or improve tissue function or a whole organ [3]. This approach utilizes cells, biomaterial scaffolds, and signaling molecules for the repair of diseased or damaged tissues. In the TERM strategies, the development of a man-made/synthetic ECM is a critical issue, since we need to learn how to engineer biomaterials that will help in recapitulating the early events of morphogenesis [4]. Currently, biomaterial scaffolds are designed to support cell and tissue growth, aiming at a macroscopic level to be compatible with the mechanical loading of the surrounding organs and tissues. However, to maintain the proper cell phenotype in an engineered biomaterial scaffold, it may be necessary to recreate the complexity and hierarchical organization observed in natural ECM, seeding/infiltration of cells into the biomaterial scaffold, and culturing the seeded scaffold with adequate nutrient supply [5].
Natural ECM materials provide physiologically relevant cellular environments, as they are a rich source of bioactive molecules. Therefore, those natural ECM-origin materials have been widely studied to engineer ECM analogs. However, to understand the molecular and biophysical mechanisms by which the ECM analogs elicit diverse effects on cellular differentiation and morphogenesis, it is crucial to use chemically and physically defined ECMs that can be reliably reproduced. In this respect, synthetic ECMs have been developed that feature defined and tunable compositions, organization, biomechanics, and ECM remodeling capabilities. Considering all these assumptions, with the present chapter, we intend to provide an updated overview on the applicability of natural materials, mainly the ones present in the ECM composition, to the processing of biomaterial scaffolds for TERM approaches.
8.1.1 Extracellular Matrix (ECM) Structure and Composition
The ECM of human tissues is a dynamic and hierarchically organized structure composed of water, proteins, and polysaccharides (such as the glycosaminoglycans [GAGs]: hyaluronic acid [HA], dermatan sulfate [DS], chondroitin sulfate [CS], heparin, heparan sulfate [HS], and keratan sulfate [KS]), proteins (such as collagen, elastin, fibronectin, and laminin), and proteoglycans (PGs) (including aggrecan, brevican, decorin, keratocan, lumican, neurocan, perlecan, syndecans, and versican) synthesized by the adjacent cells [6–8]. In this complex structure, the collagen fibers provide strength to the tissue and, more importantly, have many cell-adhesive peptide moieties intended to allow for cellular anchoring. This hydrated gel composed of PGs and other proteins fills the extracellular space, creating an appropriate microenvironment for ensuring the tissue maintenance and remodeling by cells in response to appropriate stimuli, while allowing for the diffusion of nutrients, metabolites, and signaling molecules. These components interact to form an interconnected nano- or microranged fibrous network bound to the membranes of cells. Indeed, tissue ECMs act as a scaffold to support and hold cells together, to control their structure, and to regulate cellular functions like adhesion, migration, proliferation, differentiation, and ultimately, tissue morphogenesis [8,9]. The ECM also serves as a storage depot and a controlled release system for growth factors and signaling molecules.
The ECM interacts with the adjacent cells both mechanically and chemically, remodeling the architecture of the tissues. The structure of different collagen types within the ECM determines its function as a structural element of the connective tissues [6]. Tendon ECM, for example, is composed of parallel and aligned collagen fibrils, while those found on the skin are mesh-like. In most connective tissues, the matrix macromolecules are secreted by fibroblastic cells into the extracellular space. In specialized types of connective tissues, such as cartilage and bone, cells of the fibroblast family (chondrocytes and osteoblasts, respectively) are responsible for ECM deposition. The matrix either becomes calcified into the hard and tough structures of bone and teeth, or can form the transparent matrix of cornea. ECM can also adopt the cord-like organization that gives tendons their tensile strength and elasticity.
8.1.2 Fundamentals of Scaffolding Using Naturally Derived Materials
Efforts in the area of TERM have been directed to produce biomaterial scaffolds, which physically support cells and providing conditions for cell adhesion and growth, mimicking the native ECM of tissues [10]. Those scaffolds can be obtained from different materials, including biodegradable polymers, that is, synthetic or natural in origin, ceramics or composites containing both polymer and ceramic phases. Generally, those systems aim at being resorbable under physiological conditions. The degradation kinetics of an ideal scaffold should follow the tissue growth kinetics, in such a way that the material is completely degraded when the tissue is fully regenerated [3]. Moreover, appropriate biocompatibility, porosity, pore size, surface properties, and mechanical stability have been defined as being critical requirements of a biomaterial scaffold [11,12]. The biomaterial scaffold biocompatibility relies on the nontoxic effect of both the scaffold and of its degradation products, to ensure device safety [13]. Another requisite is the total scaffold porosity and the dimensions of the pores, in order to obtain enough surface area for cells attachment and consequent proliferation. The interconnectivity of those pores is a critical issue in ensuring opportunities for cell migration and further colonization of the scaffold surface. Furthermore, pore interconnectivity allows the scaffold to maintain cell viability by enabling diffusion into the scaffold of required nutrients and oxygen, and diffusion out of metabolic residues. The chemistry/bioactivity of the scaffold surface is important to provide the right cues for the cells to secrete ECM components, to allow the ECM to mature and to facilitate cellular remodeling of the construct. Another important aspect contributing to the efficacy of the biomaterial scaffold in mimicking the functionality of the natural ECM is the balance between the level of porosity and the mechanical properties [11]. Ideally, the mechanical properties should provide a good and stable integration of the populated construct with the surrounding host tissues to sustain the stresses applied on implantation. Ultimately, all these scaffolding requirements will drive the cells to build up fully functional adult tissues.
8.2 Naturally Derived Materials
As previously mentioned, natural ECM is mainly composed of collagen, arranged in a hierarchical manner with laminin, fibronectin, and PGs in a complex topography at the nanometer range [14]. Three-dimensional (3-D) natural ECM biomaterials provide physiologically relevant cellular environments, as they are a rich source of bioactive molecules, so they have been widely studied to engineer tissues. ECM protein such as collagen or chitosan are very good candidates for these approaches and can be modified for specific cell types [14]. Natural-origin biomaterials also allow the diffusion of soluble molecules to the basal and apical surface [14]. These polymers are often combined with other natural or synthetic biomaterials to produce scaffolds for TE applications (Table 8.1). The following sections describe the origins and applications of many naturally derived materials.
Table 8.1 Biomaterials Used in Tissue Engineering and Regenerative Medicine Approaches Derived from Different Natural Sources






8.2.1 Animal Origin
8.2.1.1 Collagen
Collagen is the most abundant protein in animals, providing the main structural and mechanical support to the tissues such as cartilage, bone, and teeth [14]. This protein also forms molecular strands that strengthen the tendons and strong sheets that support the skin and internal organs [15]. Among the different collagen types, type I collagen is the most abundant component of the ECM and can be used as scaffolding material, promoting cell migration, wound healing, and tissue regeneration [16]. Collagen may be used for TE in the following applications: artificial skin, tendons or blood vessels, bone graft substitutes [17], dental [18], corneal, or stress urinary incontinence implants, and regeneration of nerve [19], cartilage [20], skin, or other organs [16]. Collagen-based biomaterials can be prepared from two fundamental techniques. One is to decellularize the collagen matrix, preserving the original tissue shape and ECM structure, while the other relies on extraction, purification, and polymerization of collagen and its components to produce a functional scaffold.
In a recent work, collagen type I scaffolds were produced by combining gel and electrospinning technologies to create a novel 3-D hybridized collagen implant with an aligned ultrastructure, aiming at tendon regeneration. The construct was implanted in rabbits, and results showed that the implanted construct was biodegradable, biocompatible, and possibly could be considered as a substitute for allografts in the near future [21].
Collagen-based scaffolds have been extensively used in clinical practice, such as Chondro-Gide® (Geistlich Pharma AG, Wolhusen, Switzerland), aimed at repairing cartilage defects [22]. Short-term results are very promising, however, long-term follow-up studies are needed to determine if the grafted area will maintain structural and functional integrity over time [22].
8.2.1.2 Fibronectin
Fibronectin has several important functions in the ECM, such as providing structural support and signaling cues for cell survival, migration, differentiation, and growth [23], as well as inducing cell attachment and spreading through its cell binding sites and related synergy sites [24]. Fibronectin exists in a soluble form in the plasma and in an insoluble, fibrillar form in the ECM [25]. During tissue repair, fibronectin is converted into biologically active ECM fibrils through a cell-dependent process [25]. Fibronectin-based scaffolds and hydrogels have been used for different TE applications such as spinal cord injury [26] or to improve the functionality of other biomaterials [27].
Fibronectin matrix mimetic variants were designed and analyzed for their ability to support new ECM assembly. The ability of fibronectin matrix mimetics to direct cell–substrate interactions and regulate ECM assembly was demonstrated, making these variants promising candidates to be used as bioactive surfaces [25]. Moreover, with the objective of mimicking normal epithelium regeneration in a synthetic scaffold, in vitro, poly (L-lactide-co-caprolactone) (PLLC) was processed into a nanofibrous scaffold using electrospinning technology, and fibronectin was engrafted onto the scaffold fibers. This scaffold was found to greatly promote the epithelium regeneration [28].
8.2.1.3 Hyaluronic Acid
Hyaluronan, also named HA or hyaluronate has been extracted from bovine vitreous humor, rooster combs, or umbilical cords [29]. HA is an important polysaccharide naturally present in the human body and it has various biological roles in human tissues. It is a GAG component of connective tissue, synovial fluid, and in the vitreous humor of the eye [29]. In cartilage, for example, HA organizes the ECM into a strong structure via assembling the large PG aggrecan [30]. It is a highly biocompatible polysaccharide and is often used for biomedical applications including cartilage [31], bone [32], or viscous supplementation in joint diseases [33]. HA injection is a common treatment for osteoarthritis [34], as HA molecules can elicit pro- and anti-inflammatory responses depending in its molecular weight. HA also plays important roles in protein adhesion and provides attachment sites between the chondrocytes and the ECM of articular cartilage [35].
HA-based TE strategies have been used extensively in clinic. For example, the Hyalograft®C (Anika Therapuetics, Bedford, MA) membrane has been used to repair cartilage defects. In a study using this membrane and the technique of matrix-induced chondrocyte implantation (MACI), patients were followed after implantation during 2 years, by magnetic resonance imaging [36]. The postoperative observations showed dynamic processes in cartilage repair over time, with positive effects. Another report of a 5-year follow-up of the MACI technique also shown good results: 8 out of 11 patients rated the function of their knees as much better or better than before the surgery [37].
8.2.1.4 Fibrin
Fibrin is not a regular component of the ECM but it exists as a temporary matrix that will be replaced by ECM [38]. Fibrin is the result of fibrinogen polymerization in the presence of thrombin [39]. Its main biological functions are blood clotting, fibrinolysis, cellular and matrix interactions, inflammation, and wound healing [40]. Fibrin is generally used in form of gels [41], glue [42], or patches [43]. Fibrin hydrogels for cartilage TE are usually seeded with chondrocytes to produce composite constructs cultured in vitro or in vivo to generate cartilaginous matrix [44]. The major disadvantages of fibrin hydrogels include the poor mechanical properties and rapid enzyme-catalyzed degradation [30].
Recently, a 3-D fibrin-based patch was developed for cardiac TE applications. Authors have successfully engineered a human cardiac tissue patch starting from human embryonic stem cell (hESC)-derived cardiomyocytes. The structural and functional properties of these patches provide the closest in vitro approximation of native human heart tissue [45].
8.2.1.5 Proteoglycans
PGs, including CS, DS, HS, and KS, comprise a core protein to which GAG chains are covalently linked, and are an important structural family of macromolecules found in the ECM [46]. They are one of the major classes of natural compounds found in the ECM that convey important properties to cells, connective tissues, and basal membranes [47]. Cell-surface PGs and ECM are involved in cell signaling, proliferation, adhesion, and motility [48]. PGs have been applied in TE mostly in scaffolds (matrigels and collagen–CS matrices), where PGs or their GAG chains are incorporated into the scaffold to promote cell growth, tissue remodeling, and intracellular signaling [48].
CS has been isolated from natural sources such as bovine cartilage [49], chicken cartilage [50], sturgeon [51], shark, and ray [52]. Both CS and DS chains have interesting functions in the development of the central nervous system, wound repair, infection, growth factor signaling, morphogenesis, and cell division [53]. It is normal to perform a CS cross-linking treatment with other polymers such as gelatin or collagen to increase the stability of the materials [54,55]. A study was performed to evaluate the efficiency of intra-articular injection of CS carried by a hydrogel in the treatment of chondral defects in adult rabbit models [56]. The biomechanical and histological properties of the repaired cartilage were both improved by this hydrogel [56].
DS can be isolated from marine species such as ray skin and the body of clams [57]. DS can act as a stabilizer, cofactor, and/or coreceptor for growth factors, cytokines and chemokines, a regulator for enzyme activity, or as a signaling molecule in response to cellular damage such as wound or infection [53]. Recombinant DS peptidoglycosaminoglycans have been studied for TE, showing that they are able to modulate the structure of other biomaterials, for example, collagen, thus aiding in the cellular adhesion [58]. Likewise, DS incorporated into alginate/chitosan microspheres, helped to stimulate cell proliferation [59].
HS is also a member of the GAG family and its structure is related to that of heparin [60]. HS is usually used to describe heparin-like by-products of the industrial preparation of heparin from animal tissues such as bovine fetal rib growth plate [61]. HS is found in all animal tissues as a PG, in which two or three HS chains are attached in close proximity to cell surface or ECM proteins [62]. HS functions are related to interactions between ECM components essential for the control of cell proliferation, differentiation, adhesion, and migration [60]. HS is normally used for cross-linking with other biomaterials [63] or immobilized in a scaffold [64]. HS conjugated with chitosan has been used for preparing nanoparticles for osteogenic differentiation induction, with promising results [65].
KS is another type of GAG that comprises different forms present in diverse tissues, including articular cartilage, reproductive tissue, and neural tissue [66]. It has been isolated from bovine cornea [46].
8.2.1.6 Silk
Silk is the building element of many arthropod nets, cocoons, and prey traps [67]. Silks are a family of structural proteins that are biocompatible, degradable, mechanically superior, and that can be chemically modified for a vast range of biomedical applications [68,69] such as cornea [70] or bone [71].
Fibroin is one of the two main kinds of silkworm silk protein, and has been investigated for biomedical applications. It presents high biocompatibility, biodegradability, limited inflammatory response, and excellent mechanical properties [67,69]. Silk polymers have a side chain that can be used for binding to globular proteins and growth factors, which has been exploited to produce a platform for precursor cell differentiation [14].
The efficiency of a silk fibroin-based biodegradable material to generate small diameter grafts was evaluated. The graft was implanted in rat abdominal aorta, and it showed excellent long-term patency and optimal mechanical properties. Endothelial and smooth muscle cells migrated into the graft after implantation and organized into endothelial and medial layers, generating a vascular-like structure [72].
8.2.1.7 Chitosan
Chitosan is a natural polymer that has interesting properties for TE applications. It is biodegradable, biocompatible, and has many structural similarities to GAGs [73]. Chitosan is obtained from chitin, the second most abundant polysaccharide (after cellulose), which is the major element of the shells of many crustaceans, such as shrimps or crabs [73]. Chitosan is obtained by a de-N-deacetylation (DD) of chitin [73]. This process is usually made in alkaline conditions, resulting into chitosans with different degrees of DD and, thus, different molecular weights [74]. The source of chitosan and the chemical modification may influence both parameters. The DD has been shown to be important for cell biocompatibility, attachment, and growth [75]. One of the interesting properties of chitosan is its ability to be processed into porous structures, which support cell growth and ECM deposition [76]. Additionally, chitosan is reported to degrade in vivo mainly by enzymatic hydrolysis [74,77]. Lysozyme, one enzyme present in the human body, has been used to enhance the formation of pores in situ, in chitosan scaffolds coated with calcium phosphate [78].
Chitosan-based scaffolds have been studied for many TE applications, such as nerve regeneration [79] or cartilage repair. In this tissue, chitosan-based scaffolds promoted cell proliferation [80], differentiation [81], and metabolic activity [82], as well as mesenchymal stem cell (MSC) chondrogenesis in vitro [83] and in vivo [84]. Chitosan has been explored for preparing hydrogels, namely a very promising gene-activated chitosan–gelatin matrix [85]. The referred matrices were capable of releasing, in a controlled fashion, transforming growth factor beta 1 (TGF-β1), and promoted chondrocyte proliferation. When seeded with human articular chondrocytes (hACs), this injectable hydrogel was able to regenerate and repair a lesion made in bovine articular cartilage, showing the great potential of this novel cell delivery system for cartilage TE [86].
8.2.2 Plant Origin
8.2.2.1 Cellulose
Cellulose is the most abundant naturally occurring polymer of glucose, found as the main constituent of plants and natural fibers such as cotton and linen. Cellulose is also produced by some bacteria, Acetobacter xylinun, being the most well studied [87]. Gluconacetobacter xylinus, another type of bacteria, is one of the most efficient producers of cellulose [88]. Both types of celluloses (plant and bacterial) are chemically identical, a polymer of β-D-glucose units, which accounts for its high crystallinity and its insolubility in water and other common solvents. Cellulose is degraded by cellulases existent in bacteria and fungi, not present in the human body. It is also to some extent degraded by hydrolysis, although highly resistant, due to its compact structure.
Plant-derived cellulose has not been explored much in the biomedical field, since the human body is not able to degrade it. One of the few reports consists of the development of a thin film using a layer-by-layer technique, combining anionic rod-like cellulose nanocrystals with chitosan [89].
8.2.2.2 Starch
Starch is the main carbohydrate reservoir of higher plants, found in storage organs, such as grains of maize and rice or the tubers of cassava and potatoes, present in human diet. Increasingly, starch is also used as a renewable raw material, as a source of energy after conversion to ethanol, and for many different industrial applications [90]. Starch is a relatively simple polymer composed of amylose (20–30%) and amylopectin (70–80%). Amylose is a linear polysaccharide of several thousand units of glucose linked together by (1 → 4) bonds. Amylopectin, consist mainly of (1 → 4) linked glucose residues, is a branched molecule with (1 → 6) linkages at every 25–30 glucose units distance [91].
Different relative weight percentages of both molecules are present in different types of starch, exhibiting significant differences in terms of properties [92]. For instance, for most cereal starches, the relative weight percentages are 18–33% of amylose and 72–82% of amylopectin. Starch is a semicrystalline, with a degree of crystallinity between 15% and 45% [91].
The original application of starch was in the food industry, as a food additive. In its native form, it is used as thickener, when heated in water, the helices within the amylopectin of starch melt and the granule starts to swell, increasing the viscosity of the solution [93]. Starch is also used in the paper industry, where it is used as a filler to give the strength to the final paper sheet. It is also used in paper coatings to improve quality [90]. Starch is enzymatically degraded by α-amylase, which exists in human blood serum [94].
In the biomedical field, Reis et al. [95] have developed extensive work concerning the investigation of several blends of corn starch with synthetic polymers namely: polyethylene-vinyl alcohol (SEVA-C); cellulose acetate (SCA); polycaprolactone (PCL), and polylactic acid (SPLA). These starch blends showed to be biocompatible both in vitro [96,97] and in vivo [98]. Starch blends can be processed by thermoplastic techniques into different shapes, such as microspheres [99], 3-D porous scaffolds [100–102], and hydrogels [103]. Starch microspheres were used to release growth factors to enhance osteogenic differentiation [99]. Osteoblast like cells adhered and proliferated in SEVA-C scaffolds processed by injection molding technology [104]. Furthermore, the same materials and also its composite with hydroxyapatite, showed to be biocompatible in vitro, as well as in vivo in an intramuscular and intracortical model in goats [96]. Another study using the same composition of SEVA-C coated with a biomimetic calcium phosphate layer, and SCA scaffolds produced by extrusion with blowing agents implanted in femurs of rats, showed bone formation [101].
Starch combined with polycaprolactone (SPCL) scaffolds, cultured with bovine articular chondrocytes, showed enhanced cartilaginous matrix [105,106]. This same blend also evidenced good results with bone marrow stromal cells (BMSCs) differentiated into osteogenic lineage [100]. It has also demonstrated that endothelial cells (ECs) grown on SPCL scaffolds display an adequate phenotype and genotype, which indicates the angiogenic potential of these scaffolds [107,108].
SPCL scaffolds combining SPCL micro- and PCL nanomotifs, respectively produced by rapid prototyping (RP) and electrospinning techniques showed to be suitable for osteoblasts like cells [102].
8.2.3 Algae Origin
8.2.3.1 Alginate
Alginates are polysaccharides isolated from brown algae such as Laminaria hyperborea and Lessonia [109]. Alginate gels are used in several applications, ranging from the food industry to pharmaceutical manufacturing [110].
In the biomedical field, alginate hydrogels have been used for drug delivery. anti-cancer drugs, such as daunomycin [111], or the combination delivery of several drugs, such as methotrexate, doxorubicin, and mitoxantrone, using different release methods [112], has been achieved. Growth factors were also incorporated in alginate hydrogels [113–116]. For instance, alginate beads with vascular endothelial growth factor (VEGF) incorporated were shown to stimulate ECs in vitro
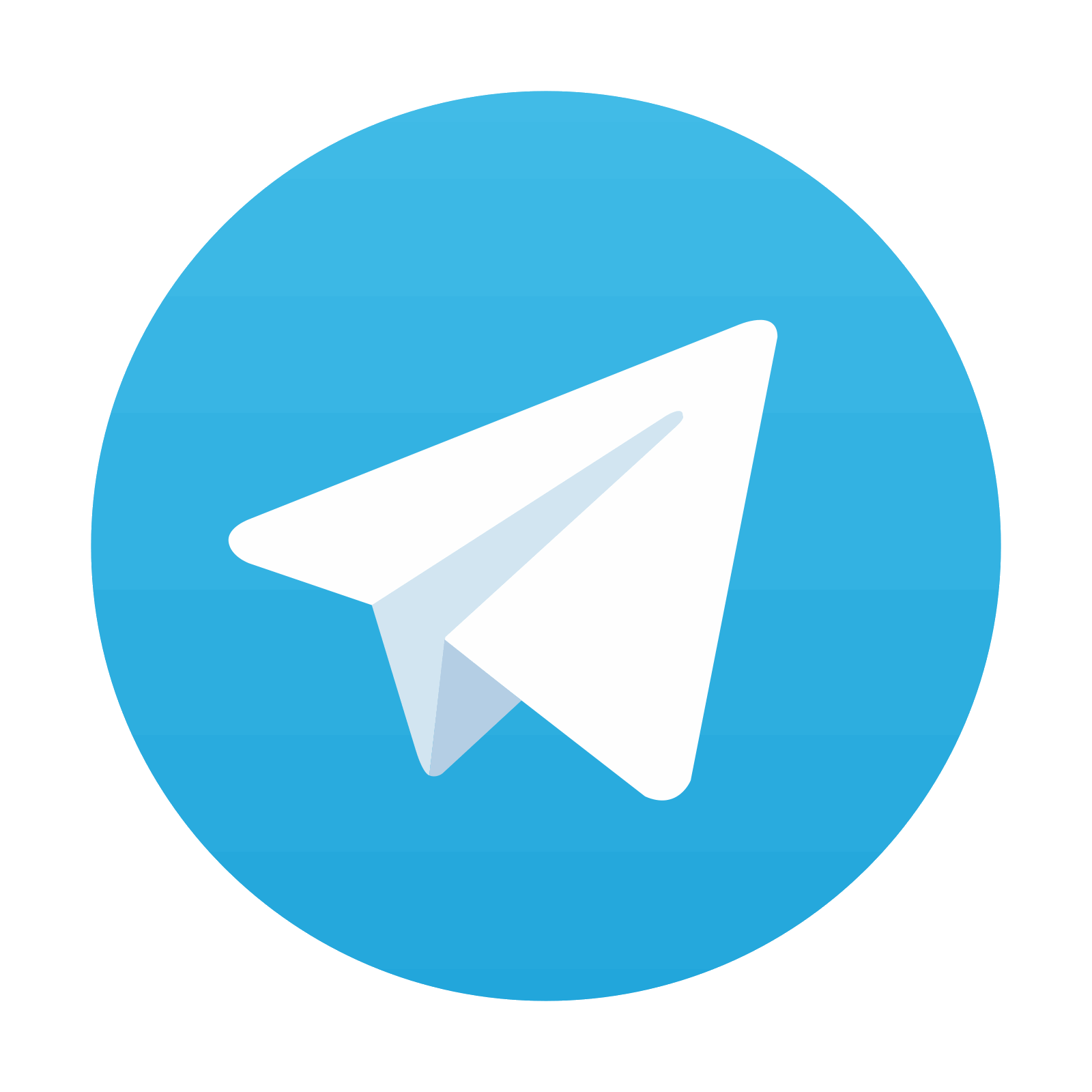
Stay updated, free articles. Join our Telegram channel
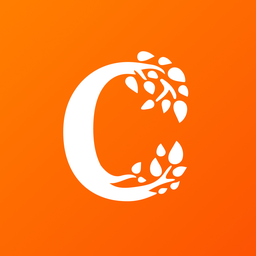
Full access? Get Clinical Tree
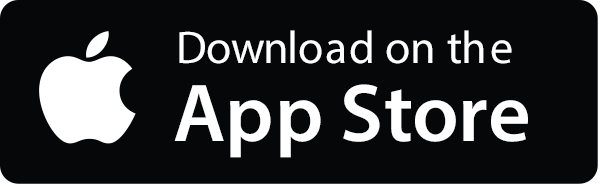
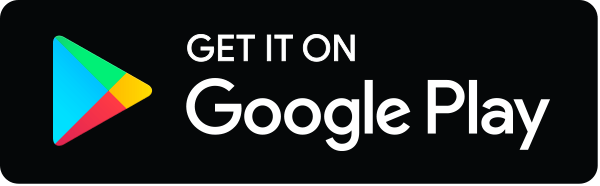