Engineering the Mechanical and Growth Factor Signaling Roles of Fibronectin Fibrils
Department of Biomedical Engineering, Virginia Commonwealth University, Richmond, VA, USA
6.1 Introduction
The in vivo presentation of extracellular matrix (ECM) plays several critical roles in cell migration, survival, differentiation, and organization. The ECM provides a complex presentation of cell attachment sites, mechanical signals, and tethered growth factor signals. However, the effects of this complex presentation is often ignored in tissue engineering and regenerative medicine, oftentimes replaced by surfaces coated with adsorbed ECM molecules, tethered ECM protein fragments, or tethered peptides that contain sequences that facilitate cell attachment. These approaches fail to replicate the complex environment that cells would be exposed to in vivo. In this chapter, we will discuss the mechanical and signaling roles of one particular ECM protein, fibronectin (FN). FN is a soluble protein that is found at high concentration in the blood plasma [1]; it is stretched by cells into an extended conformation that is purported to expose cryptic binding sites to facilitate assembly of insoluble fibrils [2]. FN assembly is required for collagen assembly [3–5], fibrillin assembly [6], and tenascin assembly [7–9], and as such, is crucial to successful tissue engineering. We will first discuss the mechanisms of FN fibrillogenesis as well as the roles of FN fibrils in mechanotransduction, cell attachment and migration, and growth factor signaling. Subsequently, we will discuss in vitro mechanisms to assemble FN fibrils and highlight examples of FN matrix signaling in several tissue engineering applications.
6.2 Structure of Fibronectin
FN is a dimer of a 250-kDa protein that forms insoluble fibrils that are a critical component of ECM [10,11]. It is made up of a series of three domain types connected end to end [12,13] (Figure 6.1). The N-terminus of FN consists of nine FN-I and two FN-II domains, each of which contains an internal disulfide bond [12,14]. The C-terminus of FN consists of three FN-I domains and contains intermolecular disulfides that link the two FN monomers to form the dimer. The central portion of the FN molecule is made up of 15 tandem FN-III domains; these domains each consist of seven beta strands folded into a beta-sandwich structure and contain no internal disulfides [15,16]. Numerous studies have shown that these domains are capable of unfolding under both chemical and mechanical forces [17–19]. Interestingly, these domains have nearly identical structure, but have only roughly 30% sequence homology [20,21]. The significant variation in domain sequence most likely gives rise to the wide variance in chemical and mechanical stability that is observed between the domains [17,19,22,23]. FN in solution adopts a compact conformation in which the 2nd–4th FN-III domains (III-2–4) of one subunit interact with the 12th–14th FN-III domains (III-12–14) of the other, causing the FN dimer to fold onto itself [24].

6.3 Assembly of Fibronectin Fibrils
FN is present in blood plasma at extremely high concentration in a soluble state. Cells bind to FN and stretch it by applying contractile forces. This stretching causes FN to assemble into insoluble, rope-like fibrils [25–28]. These fibrils are an extremely unique biopolymer, in that they are highly elastic and can be stretched to up to four times their resting length. The existing dogma for FN assembly is that cell-applied contractile forces stretch FN to expose a cryptic binding site; however, several studies over the course of 40+ years of research have yet to identify such a buried site. It is, however, well documented that FN assembly requires the application of cell-generated forces [2,29,30], and that FN is absolutely necessary for tissue formation; deletion of the FN gene is lethal at the embryonic stage in mammals [26]. Previous studies have demonstrated that the 70-kDa N-terminal fragment, which composes the nine FN-I and two FN-II domains, is necessary for FN fibrillogenesis; however, this 70-kDa N-terminus is not a cryptic FN–FN attachment site. The 70-kDa fragment is readily exposed in soluble FN [31–33], and as such, could not be a buried cryptic binding site. While a cryptic binding site has not been discovered, several studies have shed light onto potential mechanisms of FN assembly. Mosher and colleagues have shown that a domain from the streptococcus pyogenes protein adhesin F1 is capable of binding to the 70-kDa fragment of FN through beta-strand addition [34–36]. The 15 type III domains are made of seven beta-strand sandwiches, which have several features that block beta-strand addition, including twisting of the free-edge beta strands and orientation of side chains that sterically hinder addition of new beta strands [37]; however, steered molecular dynamics studies have indicated a stable intermediate of stretched type III domains in which the beta-strand-blocking features of the type III structure are removed [38,39]. Thus, it is feasible that cell-generated forces untwist type III domains and transition the domains to a stable intermediate that is capable of beta-strand addition with the 70-kDa N-terminus of new FN molecules (Figure 6.2). This could offer a possible explanation for the elusiveness of the cryptic FN–FN site; since each of the 15 type III domains shares a similar structure, it is possible that all 15 domains are capable of binding new FN molecules when exposed to cell-generated tension. This would also suggest an extremely elegant, mechanosensitive assembly process. Given the previously discussed wide array of mechanical stabilities of the 15 type III domains, one could imagine a mechanism in which FN assembly is highly controlled by the magnitude of applied cellular forces; small forces would open only the weakest domains, generating only slight fibril assembly, while large forces would open many domains and drive significant fibril assembly.

6.4 Mechanics of Fibronectin Fibrils
While the exact mechanism of FN fibril formation is still unknown, we do know that insoluble FN fibrils are highly elastic structures and can be stretched to up to four times their resting length [40–43]. This elasticity requires cell contraction; disruption of cytoskeletal contraction relaxes stretched fibrils [41]. Two different mechanisms have been proposed to explain the elasticity of FN matrix fibrils [44]. One theory contends that the elasticity is a function of FN-III domains unfolding under tension [45,46]. These domains contain no internal disulfides and can be mechanically unfolded by atomic force microscopy [17,18]. It is unclear how relevant these atomic force microscopy (AFM) pulling forces are to the in vivo assembly of fibrils; the unfolding by AFM occurred at high forces on the order of 100 pN, due to high pulling rates. In cell-assembled fibrils, the pulling speed is nearly zero, so it is difficult to extrapolate the cell-applied force that would be needed to unfold these domains. In addition to mechanical unfolding of FN-III domains, transient opening of certain FN-III domains has been demonstrated in the absence of applied force during the assembly of in vitro assembled super-FN [47,48]. The second theory to explain fibril elasticity contends that FN molecules are in a compact conformation in relaxed fibrils, and are stretched to the extended conformation in elongated fibrils [49]. These two mechanisms are not exclusive; elasticity of fibrils may be a combination of the two. Recent studies have suggested that a subset of the type III domains are stretched open in cell-assembled fibrils [19]. Unfolding of this subset cannot explain the fourfold extensibility; these domains would have to be completely extended in order to generate fibril extensions on this order, and forces needed to completely extend the domains are greater than the forces generated by attached cells.
Regardless of the mechanism, the fourfold extensibility of FN fibrils is extremely significant in cellular mechanosensing. There are a great number of recent studies that have shown that cells are extremely sensitive to the elastic modulus of the substrate to which they are attached: substrate rigidity regulates cell spreading [50], cell migration [51–55], cell survival [56–58], and cell differentiation [54,59–65]. The mechanism by which these mechanical signals is transduced to intracellular signaling pathways has yet to be elucidated; however, the majority of cell lines investigated in these studies are known to assemble FN fibrils in vitro. For example, human mesenchymal stem cells (hMSCs), which have been well characterized as exhibiting stiffness-dependent differentiation, assemble substantial FN fibrils (Figure 6.3). The presence of these fibrils not only has the potential to alter growth factor signaling (discussed in further detail below), but they also may serve to alter the ability of cells to generate contractile forces. Odde and colleagues have previously demonstrated that cells can generate larger contractile forces on soft surfaces, and they have explained this phenomenon through the use of an elegant computational model [66]. In this model, the myosin–actin adhesion-substrate system is modeled as a series of elastic spring elements that are capable of rupturing at the adhesion-substrate interface. On stiffer surfaces, the model predicts what the authors refer to as a frictional slippage regime, where the substrate stiffness results in frequent rupture events that prevent the establishment of large contractile forces. On softer surfaces, the deflection of the substrate reduces the frequency of adhesion-substrate rupture events, which allows for the generation of larger cell forces (referred to as a load-and-fail regime by the authors, as the model predicts the generation of large forces that are interspersed with infrequent but large rupture events). Assembly of FN fibrils may serve as an intermediate spring in this model; that is, the fibril would act as a spring between the substrate and adhesion. The fourfold extensibility of the fibril would thus create a situation in which cells are capable of generating large forces, even in the presence of a stiff surface. Thus, assembly of FN fibrils may facilitate larger cell forces, which have been shown to correlate with differentiation and migration.

6.5 Role of Fibronectin Fibrils in Cell Attachment
Assembled FN is not just a passive scaffold to which cells attach. It also triggers a wide array of signal cascades within the attached cells that regulate cell proliferation, migration, contractile force generation, and further ECM remodeling. FN binds to the transmembrane proteins, β1 integrin and β3
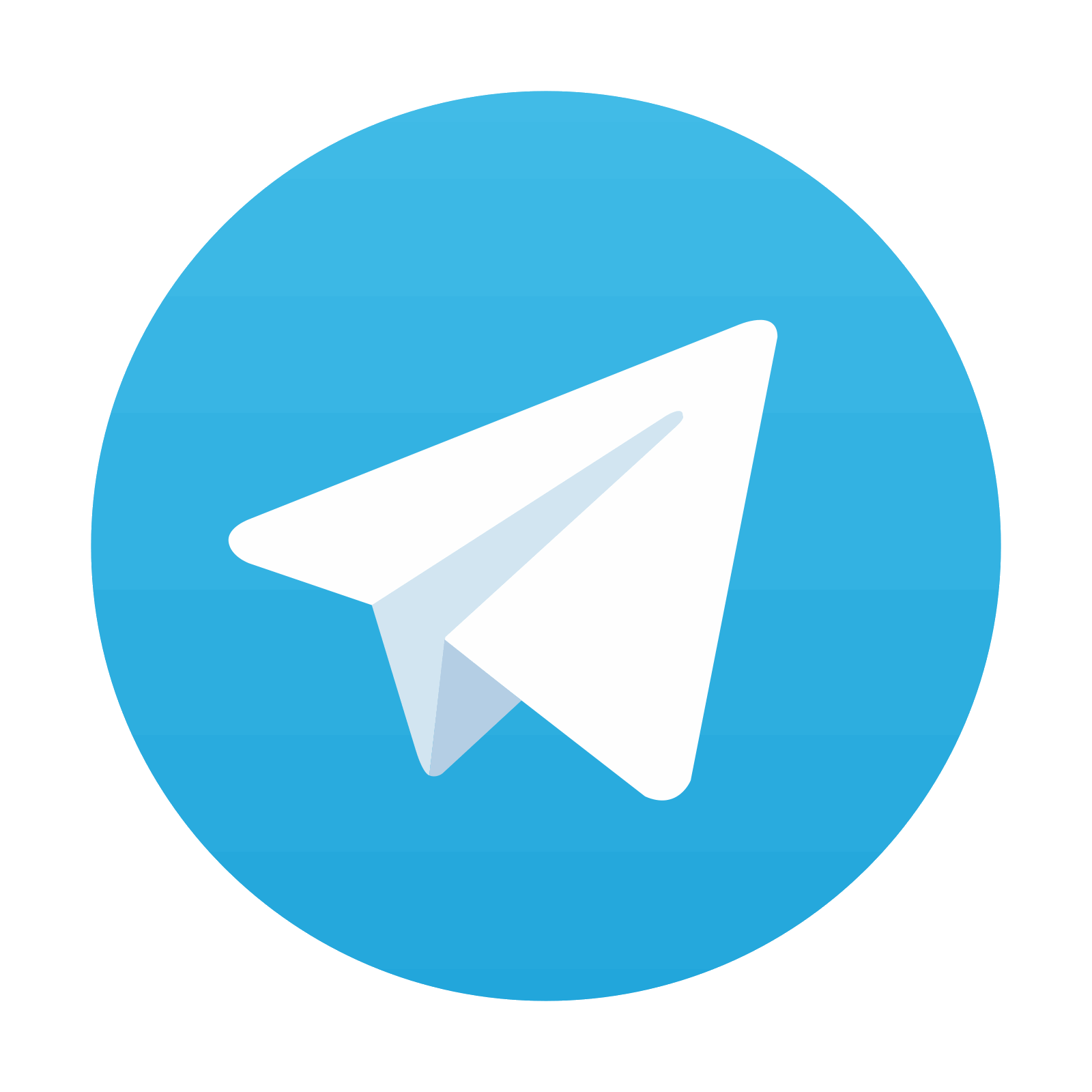
Stay updated, free articles. Join our Telegram channel
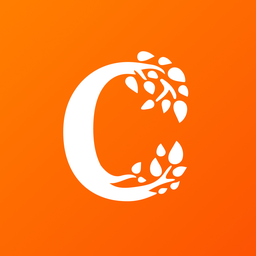
Full access? Get Clinical Tree
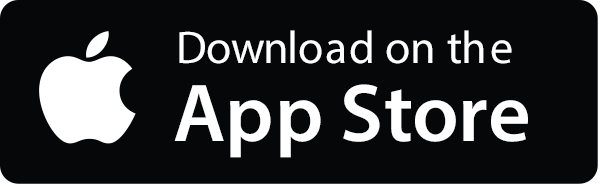
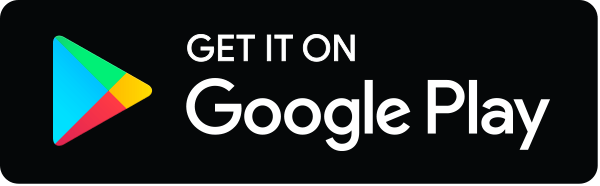