Cellular Responses to Bio-Inspired Engineered Topography
Sharklet Technologies, Inc., Aurora, CO, USA
J. Crayton Pruitt Family Department of Biomedical Engineering, University of Florida, Gainesville, FL, USA
Department of Materials Science and Engineering, J. Crayton Pruitt Family Department of Biomedical Engineering, University of Florida, Gainesville, FL, USA; Sharklet Technologies, Inc., Aurora, CO
5.1 Introduction
Biological tissues are composite materials that exhibit hierarchical structure on size scales ranging from nanometers to centimeters. A closer look at these structures reveals cells, which are on the order of tens to hundreds of micrometers, organized in distinct structures within an extracellular matrix (ECM). The ECM is a dynamic microenvironment consisting of large molecules synthesized by cells that provides biochemical cues through both presentation of adhesive ligands and sequestration, storage, and presentation of soluble regulatory molecules [1]. It also imparts biophysical cues via cellular interactions with protein structures. Cell-secreted proteins such as collagens and elastins provide physical support for cell anchorage, while proteins such as fibronectin and laminin present biochemical adhesive signals. While both biochemical and biophysical cues guide tissue formation, the biophysical cues sensed by cells in vivo, which range from microscale to nanoscale, are critical for guiding cell shape and orientation to form specialized structures that are adapted to the particular function of the tissue [2]. This chapter begins with a brief history of the foundational and pioneering work in investigating cellular responses to biophysical cues and then presents an overview of natural surface structures and their bio-inspired counterparts, including the fabrication and application of these surfaces. Advances in the design of biomaterials for cell culture have led to new ways for scientists and bioengineers to probe and answer fundamental questions about how cells receive information from, and respond to, their natural microenvironments.
5.1.1 Historical Introduction to Cellular Responses to Physical Cues
The first evidence that physiochemical cues such as substratum topography influence cell morphology was reported over a century ago. Harrison, in 1914, first recognized this phenomenon when he observed that fibroblasts from the embryonic nervous tissue of frogs elongated while cultured on spider silk [3]. Weiss coined the term contact guidance in 1945 to describe how cells orient, elongate, and migrate in response to structures in their microenvironment [4]. Through hundreds of experiments, Weiss and colleagues showed that neuronal cells adhered, elongated, and migrated along scratches in the surface of mica and glass fiber structures ranging from 10 to 30 μm in diameter [4,5]. Curtis and Varde subsequently investigated contact guidance, contact inhibition, and cell spreading of chick heart fibroblasts on silica substrates with varying surface topographies, and concluded that cell behavior and morphology were controlled by both cell density and substratum topography [6]. The pioneering investigations of cellular responses to physical cues primarily concentrated on grooved/ridged topographies or fibers due to both the marked cellular responses observed and the ease of fabrication. Microfabrication techniques developed in the electronics industry, such as photolithography and electron beam lithography, began to gain popularity in biomaterials research to produce cell culture substrates with micro- and nanoscaled topographies of various shapes and spatial arrangements approximately 20 years ago [7]. The application of microfabrication techniques in biomedical engineering has resulted in tremendous progress toward elucidating cellular responses to both chemical and topographic patterning, as reviewed previously [8,9]. This chapter will specifically examine the bio-inspired, engineered surface topographies that have emerged as a result.
5.1.2 Physical Cues in Nature
In vivo cells receive biochemical and biophysical cues through interactions with the ECM, soluble factors, and neighboring cells (Figure 5.1A). The ECM consists of a variety of components with specific properties that combine synergistically to form tissues. Collagen provides structural support, elastin and proteoglycans impart elasticity to the matrix, and structural glycoproteins adhere these components together. These components collectively provide mechanical support for cell anchorage, determine the orientation and shape of the cell, control cell growth, promote cell differentiation and sequester, store, and present soluble signaling molecules [10]. The ECM also facilities long-range force transfer between cells to coordinate cellular and tissue responses such as wound healing over large areas [10].

Collagen is the most abundant protein found in the human ECM. It is secreted by cells, and after modification by proteolytic enzymes outside the cell, collagen molecules can self-assemble into fibrils with diameters ranging from 10 to 300 nm [11]. These fibrils are subsequently stabilized by disulfide and other covalent cross-links that form between collagen molecules. Fibrils can then aggregate into collagen fibers that reach diameters of several microns [11]. These fibers are an integral component of the basement membrane, which is best described as the basal laminae, thin flexible mats of specialized ECM (40- to 120-nm thick) that underlie or surround many cell types, and the layer of collagen fibrils connecting it to the underlying connective tissue [11]. The basement membranes of different tissues perform specialized functions so each has its own complex nano- to microscale architectures ranging from 5 to 200 nm [9], which include structures such as pits, pores, ridges, and fibers [8]. Both the composition and the structure of ECM are highly correlated with cell phenotype and tissue or organ function. Badylak et al. illustrated the relationship between structure and function by characterizing the ultrastructure and molecular composition of porcine urinary bladder, small intestine, and liver [12]. The results demonstrate that each surface of every ECM scaffold exhibits unique structural and compositional characteristics. Here, Figure 5.1B depicts the complex fibrous architecture of the basement membrane of a porcine urinary bladder. Bladders were collected from animals (∼120 kg) at a local abattoir (Thoma’s Meat Market, Saxonburg, PA), frozen (>16 hours at −80°C), and thawed completely before use. The basement membrane and underlying lamina propria were isolated and harvested from the bladders as previously described [12,13]. The tissue was then placed in 0.02% trypsin/0.05% ethylene glycol tetraacetic acid (EGTA) solution for 2 hours at 37°C with physical agitation to detach cells from the ECM.
The introduction of micro- and nanofabrication techniques into the field of biomedical engineering has allowed researchers to create highly ordered, precisely engineered topographic cues in biomaterials. Nanotopographies are structures on the same size scale as large proteins or small assemblies of molecules, while microstructures represent large aggregates of smaller structures such as collagen fibers. Throughout this chapter, the prefix micro will refer to structures >1 μm while nano will describe signals <100 nm. The ability to replicate natural topographic cues has led to pioneering work that has clearly demonstrated that, by altering substratum topography, it is possible to change cell morphology. The presentation of topographic cues has been exploited in recent years to regulate cell function. Topographies have been shown to influence cell adhesion [14], migration [15], proliferation [16,17], protein expression [18], gene regulation [16], and differentiation [19,20]. Cell shape, cell spreading, and cytoskeletal tension have been demonstrated to have a strong influence on the lineage commitment of human mesenchymal stem cells (hMSCs) [21]. Even though these patterning techniques and resulting cellular responses are now well established, very little is known about the mechanisms underlying cell sensing within the niches these cells naturally inhabit. The goal to advance our fundamental knowledge of how cells receive and process signals from their surroundings motivates researchers to recapitulate natural cellular microenvironments. The first step in this process is the design of the bio-inspired engineered topography.
5.2 Definition of Engineered Topography
The terms used to describe a topographically modified surface are numerous. These terms include: roughness, topography, pattern, architecture, structure, and texture. They are often used interchangeably and are typically combined with dimensional prefixes such as nano and micro or the word surface. Some of these combinations are surface topography, nanoroughness, microarchitecture, and microscale surface structure. Although the prefixes define and narrow the size scale of the surface, it does not provide a distinction between the root words.
In 2006, a new nomenclature was introduced called engineered topography [22]. This term was developed to be distinguishable in both definition and design from the topographies, patterns, and structures presented in previous research and patent art relating to the effect of surface characteristics on biological responses. This is not to be confused with the manufacturing term engineered surface topography, describing machined surfaces typically created by sandblasting or grinding [23].
Engineered topography, as implied by the prefix engineered, is a designed or predefined topography. The desired shape, arrangement, and dimensions of the features that comprise the topographical surface are prescribed before a fabrication technique is even considered. An engineered topography is not the consequence of a surface modification or fabrication technique. Surface features are selected based on the consideration of a specific biological response, such as the inhibition of bacterial settlement, the increase in attachment of proteins, or the alignment of cells. When a particular cell, organism, or biological system is selected, this topography becomes a predefined engineered topography, of which the geometry, size, and arrangement of the features are tailored to elicit a specific response from the living entity targeted.
In the same year, 2006, a related term was introduced by Charest et al. called mechanical topography and is defined as “a pattern of mechanical structures with regular and specifically designed size, shape, and periodicity” [24]. This definition was introduced by the authors to distinguish these distinct surfaces from mechanical roughness that was defined as “a group of mechanical features that exhibits randomness and polydispersity in terms of size, shape, and periodicity” [24]. Thus, for a succinct definition, engineered topography can be defined as a mechanical topography tailored for a specific biological response.
An engineered topography that results from the examination of a surface in nature that influences the design is termed a bio-inspired engineered topography. It is not meant to be an exact structural and dimensional copy of the natural surface, but a design that can be tailored and adjusted on the nano-, micro-, or macroscale in order to elicit the desired biological response.
5.3 Surface Fabrication Techniques
5.3.1 Fabrication of Engineered Topography
Engineered topographies are created on the surface of material substrates, typically soft plastics, by replication of microfabricated silicon molds. Silicon molds are created using lithographic and dry etching techniques. First, a two-dimensional (2-D) pattern is designed, digitized, and printed to a photomask using electron beam (e-beam) lithography (Figure 5.2). Photolithographic techniques are then used to transfer the pattern present on the photomask to a photoresist-coated silicon wafer (Figure 5.3). Next, the patterned silicon wafer is etched to a desired depth using deep reactive ion etching (Figure 5.4). As a final step, the remaining photoresist is removed from the wafer by an oxygen plasma etch. The processed silicon wafer contains three-dimensional (3-D) features etched within the wafer surface in the designed pattern. This wafer serves as a mold for topographical replication. The replication of topographical features to substrate surfaces is accomplished via an iterative casting method. This section describes the typical materials, equipment, and methods to complete this fabrication process.



5.3.1.1 Pattern Design and Photomask Generation
A pattern consists of 2-D geometric shapes arrayed over a specified area. For standard photolithography, patterns are not to contain any dimensions smaller than 1 μm. This dimensional limitation is defined based on the commonly known resolution limit of ultraviolet (UV) photolithography (∼0.5 μm under ideal conditions). The pattern must be able to be drawn and stored in a computer-aided design (CAD) program. This is necessary so that the pattern can be uploaded to computer-controlled lithography equipment and printed on a photomask. Also, patterns must not be too complex or robust such that digitized computer files exceed 1 GB as storage and uploading becomes a problem. Most geometric patterns that follow these guidelines can be successfully fabricated into 3-D topographical features. Some examples of designed patterns are included in Figure 5.5.

Most CAD software packages can be used to generate and digitize designed patterns. AutoCAD® (Autodesk, Inc., San Rafael, CA) is useful for defining the layout of multiple patterns on a single photomask. Once complete, the electronic drawing is transferred to a computer-controlled e-beam lithography system to be directly printed to a photomask. Fabricated photomasks are typically made of quartz (∼0.1-in. thick) with a thin layer of chrome (<100 nm) on one side of the quartz surface. The designed pattern is etched within this chrome layer, such that the features of the pattern are clear (no chrome), surrounded by a dark background of chrome (Figure 5.3B).
5.3.1.2 Process of Photolithography
The process of photolithography involves the transfer of the pattern present on the fabricated photomask to the surface of a silicon wafer. Prime silicon wafers are placed in an oven at ∼150°C for at least 15 minutes to completely dry the wafers. Once cooled, hexamethyldisilazane (HMDS) is vapor deposited on each wafer for 5 minutes. HMDS promotes the adhesion of photoresist to the silicon surface.
Photoresist is coated on silicon wafers by a spin coating process, typically at 4000 RPM for at least 30 seconds, but varies by photoresist type and manufacturer. Photoresist-coated silicon wafers are then placed in an oven at ∼90°C for 30 minutes to drive out all the solvent in the photoresist. This produces a completely dry photoresist layer in preparation for UV exposure. This preexposure bake is sometimes referred to as a prebake or softbake. The photoresist manufacturer will specify the exact time and temperature of the preexposure bake.
Patterns contained on the photomask are transferred to softbaked, photoresist-coated silicon wafer using a mask aligner. The photomask was attached to the mask holder, placed above the loading stage, and aligned over the center of the wafer chuck. The silicon wafer was placed on the wafer chuck and loaded into the mask aligner beneath the mask holder containing the photomask. The wafer chuck is raised so that the silicon wafer makes direct contact with the photomask. The photoresist is then exposed through the photomask using UV light (e.g., 405 nm wavelength) for time periods less than 30 seconds as dictated by resist thickness and type. The photoresist was exposed only in the areas of the pattern present on the photomask.
The exposed silicon wafer is developed by immersion in developer solution to rinse away exposure areas of the photoresist. Exposed wafers were completely immersed and agitated in developer solution for 40 seconds, removed, and immediately immersed and agitated in deionized for 60 seconds. The wafer can then be dried with compressed nitrogen.
As a final step, the developed and dried silicon wafer is placed in an oven at ∼120°C for 30 minutes. This process is typically referred to as a hard bake. The hard bake enhances the adhesion and increases the etch resistance of the remaining photoresist to the silicon wafer.
5.3.1.3 Etching of Patterned Silicon Wafers
Patterned silicon wafers are etched to a desired feature depth using processes such as deep reactive ion etching. Deep reactive ion etching utilizes the Bosch process that allows for the creation of high-aspect ratio features. A Surface Technology Systems (STS) multiplex reactive ion etcher is a capable system for this type of etching. An example process condition for an isotropic etch is listed in Table 5.1. The etch depth is controlled by the total time of the process. Approximated etch depths using 2-μm resolution patterns for different process times are listed in Table 5.1. Once etched, photoresist is cleaned from the wafer using oxygen plasma etch on the STS system using process conditions outlined in Table 5.2
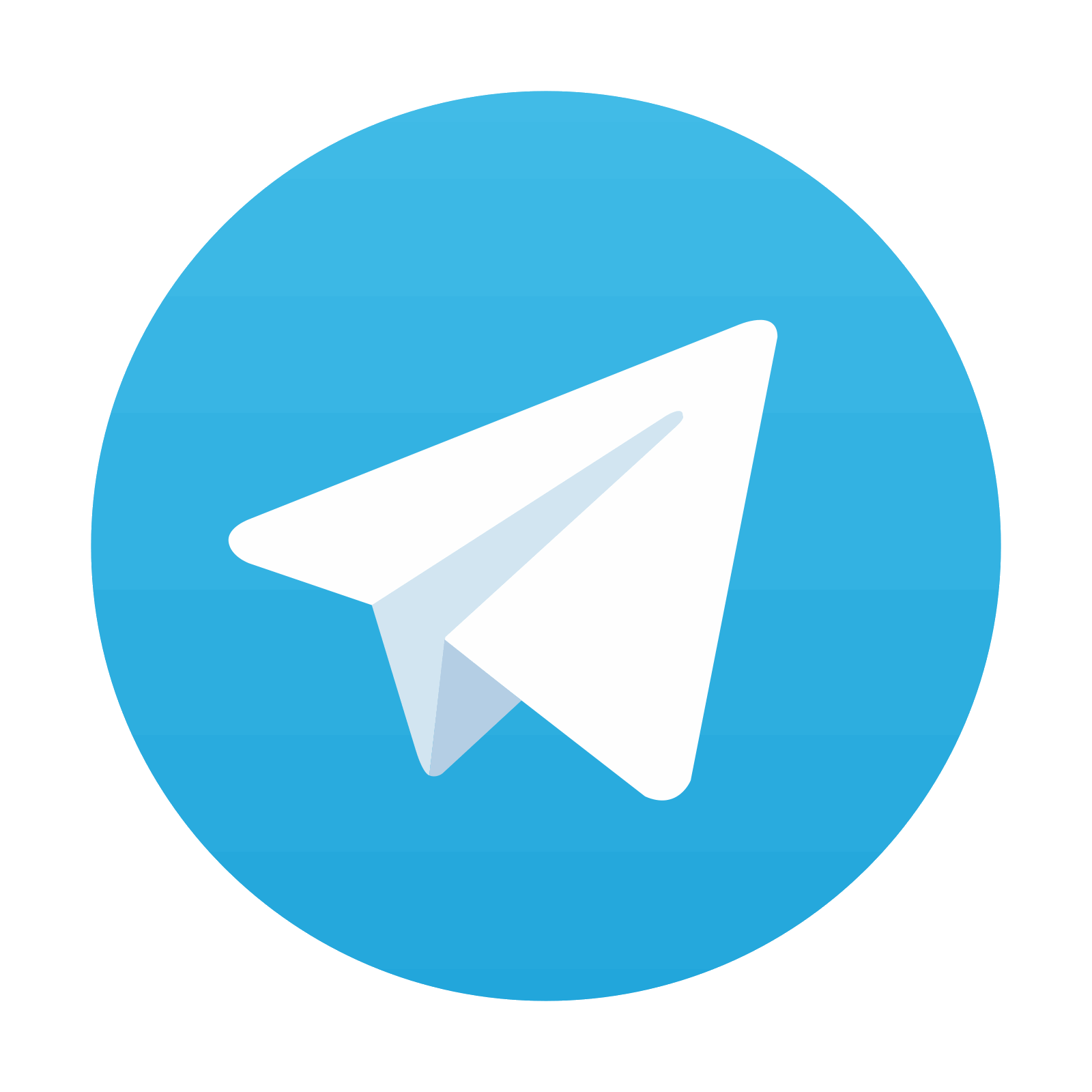
Stay updated, free articles. Join our Telegram channel
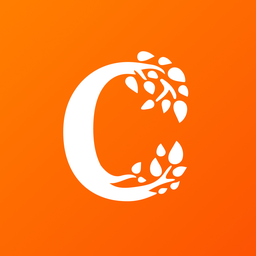
Full access? Get Clinical Tree
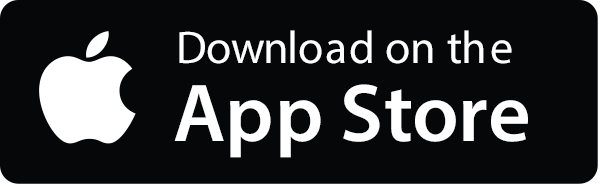
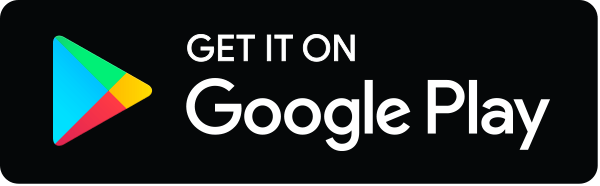