Contribution of Physical Forces on the Design of Biomimetic Tissue Substitutes
BIOMATEN, METU Center of Excellence in Biomaterials and Tissue Engineering, Department of Biomedical Engineering, Ankara, Turkey
BIOMATEN, METU Center of Excellence in Biomaterials and Tissue Engineering, Ankara, Turkey
BIOMATEN, METU Center of Excellence in Biomaterials and Tissue Engineering, Department of Biotechnology, Ankara, Turkey
BIOMATEN, METU Center of Excellence in Biomaterials and Tissue Engineering, Department of Biotechnology, Ankara, Turkey
BIOMATEN, METU Center of Excellence in Biomaterials and Tissue Engineering, Departments of Biological Sciences, Biomedical Engineering, and Biotechnology, Ankara, Turkey.
4.1 Introduction
Cells have to adhere onto biomaterial surfaces in order for the biomaterial to serve its function. Otherwise, the tissue and the implant stay as separate phases with insufficient interaction, which might eventually lead to extrusion of the biomedical devices. In order to improve the interaction, the mechanisms of cell adhesion to their environment have to be known, as the biomaterial itself cannot contribute to this interaction actively, except by presenting its chemical and physical surface to the biological entities.
4.1.1 Molecular Mechanisms of Cell Adhesion
Cells are always in contact with their surroundings and this involves communication with each other and interaction with the microenvironment. These interactions allow cells to organize in the form of tissues and function as a member of a group of cells acting in harmony. This is what differentiates the cells in an in vitro medium from those in an in vivo setting. The types of cells, the extracellular matrix (ECM) that surrounds them, the presence of nerves and blood vessels, and above all, the microarchitecture of the organ in which these cells reside define these interactions. In order to be able to communicate with their environment, the cells use a number of molecules.
The main family of transmembrane molecules involved in these contacts is called the integrins. They are positioned in the cell membrane and serve as a relay center between the cell interior and exterior. In humans, about 20 integrins are involved in the interactions with different kinds of molecules. Internally, integrins are linked to the actin cytoskeleton. Externally, integrins interact with the ECM, other cells, and the substrates such as implants. On the ECM, the main counterpart for the integrins is fibronectin, a protein with cell adhesive properties. On the cytoplasm side of the cell, the integrin molecule binds to a number of adapter proteins that connect to the actin filaments, thus, integrins do an inside–outside bridging function.
Binding between cells is achieved mainly through gap junctions, tight junctions, adherens junctions, and desmosomes. Gap junctions are a group of channels that connect the aqueous compartments of neighboring cells that allow the exchange of small molecules and ions. Tight junctions are regions of the plasma membranes fused together to form a tight seal between the cells, which does not allow transportation of ions and other solutes. Adherens junctions are more extensive connections; they connect the actin filaments of neighboring cells. Desmosomes are similar to adherens junctions in that the intermediate filaments of neighboring cells are connected. Through these interactions, the cells perform their metabolic activities and maintain homeostasis.
4.1.2 Cell Adhesion to Substrates
The main molecules involved in triggering cell adhesion to substrates belong to the integrin family. Their α and β subunits are expressed on the surface of the membranes. Each consists of a large extracellular domain, a transmembrane section, and a short tail in the cytoplasm linked to the intermediate filaments instead of actin filaments. Binding of paxillin to cytoplasmic tails has been demonstrated, and this binding is reported to regulate cell spreading, migration, and stress fiber formation (association between α4 integrin cytoplasmic tail and nonmuscle myosin IIA regulates cell migration) [1]. Integrins cluster to form matrix adhesions, which are extremely dynamic and complex structures with different sizes, compositions, and orientations. The largest, most mature structures are referred to as focal adhesions (FAs), and are involved in the attachment of cells onto substrates by way of the cytoskeleton. The β-subunit of integrin is linked through talin and vinculin to α-actinin and actin filaments, which are linked to the nuclear membrane [2]. The focal adhesion consists of an assembly of proteins including integrins, talin, tensin, vinculin and paxillin, α-actinin, Src, and the enzyme focal adhesion kinase (FAK). Vinculin and paxillin are responsible for driving cell migration, and vinculin, tensin, and talin for linkage with the actin-based cytoskeleton [3]. These adhesions and the molecules involved are in contact with the external cellular membrane through the integrins and attach to the surface of a biomaterial or ECM through fibronectin or the specific amino acid sequences on collagen such as arginine–glycine–aspartic acid (RGD) tripeptide sequences. When cells are placed on implants, they are confronted with a substrate, the architecture and the chemistry of which is completely different, and the absence of the protective ECMs of the natural tissue is a major disadvantage. The cell has to adapt to this new poor environment. If the cell in question is a stem cell, it has to differentiate into the right cell type for the targeted organ or tissue in addition to proliferation.
4.2 Physical Forces
4.2.1 Mechanical Forces
4.2.1.1 The Mechanism of Mechanical Stimulation on Tissue Regeneration
Mechanobiology investigates the mechanisms of signal transduction from the cell membrane to the cytoskeleton as well as mechanotransduction to the nucleus by way of sequential stimulation of adhesion complexes and secondary messenger proteins on actin filaments (Figure 4.1). Integrins, cadherins, and Ca2+ channels are known to be involved in the transfer of mechanical signals into the cell, which are subsequently propagated via cellular signal transduction [4]. Although the signal transduction mechanism is not understood entirely, there are studies in which integrins have been implicated in a remarkable range of mechanotransduction phenomena. Cellular responses to stretching, elevated hydrostatic pressure, fluid shear stress, and osmotic forces increase the tension on the integrins and lead to recruitment of vinculin, zyxin, and probably other focal adhesion components [5]. Multiple studies have also shown that application of strain to adherent cells triggers activation of focal adhesion kinase and c-terminal Src kinase (c-src) [6]. Mimicking the principles of mechanotransduction may become a potential strategy for tissue regeneration in guiding cells to desired phenotypes.
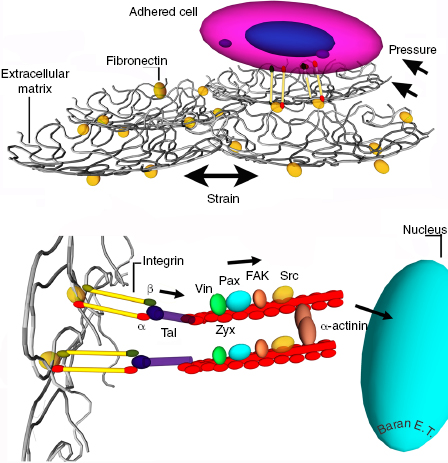
4.2.2 Thermal Forces (NIPAM)
4.2.2.1 Thermal Forces and Thermo Responsiveness in Tissue Engineering
A typical tissue-engineered product consists of a scaffold and cells and some additives such as growth factors, hydroxyapatite (HA), elastin-like peptides, and so on. A novel approach to tissue engineering is called cell sheet engineering, in which transplantable monolayers of cells are produced. To produce a cell sheet, the most commonly used strategy is to use a thermoresponsive polymer to culture the cells on. This polymer substrate changes its properties (expands or contracts) with temperature and the cell sheets that lift off are harvested [7]. Most of these thermoresponsive polymers are hydrogels. The term sol–gel transition refers to the transition between a solution and a gel form. Some hydrogels exhibit a separation from solution and solidification above a certain temperature called the lower critical solution temperature (LCST); below this temperature, the polymer is soluble. Above LCST, polymers are very hydrophobic and insoluble. For a polymer exhibiting an LCST, increasing the temperature results in negative free energy change which makes the water–polymer interaction unfavorable and the polymer–polymer interactions favorable. This is also called the hydrophobic effect [8]. Thermoresponsive polymers can be divided into two subcategories: natural polymers and derivatives (i.e., cellulose derivatives, chitosan, dextran, xyloglucan, gelatin), and synthetic polymers (N-isopropylacrylamide (NIPAM), poly(ethylene oxide) (PEO)/poly(p-phenylene oxide) (PPO), polyethylene glycol (PEG)/polyester copolymers, poly(organophosphazenes)) [9].
Many techniques are available for synthesis of thermoresponsive polymers. For example, Duarte et al. polymerized poly(N-isopropylacrylamide) (PNIPAM) by supercritical fluid foaming [10]. They also produced poly(D,L-lactic acid) (P(D,L-LA)) and P(D,L-LA)/PNIPAM foams with this technique. Biocompatibility and viability tests with L929 cells showed that these foams were highly biocompatible. This approach to production of thermoresponsive three-dimensional (3-D) scaffolds appears to be a cost-effective method of scaffold production. Kobayashi et al. designed PNIPAM surface coatings to produce cell sheets in a cell culture dish [11]. They showed that, epidermal keratinocyte sheets for use in autologous transplantation to treat skin defects, and limbal stem cell sheets for use in autologous transplantation in the treatment of corneal defects, could be produced using this technique. In another study, aminated alginate (AAlg) was copolymerized with PNIPAAm in a comb-like fashion to produce injectable hydrogels [12] and human bone marrow mesenchymal stem cells (hBMSCs) were encapsulated in them. These thermoresponsive hydrogels were shown to be not cytotoxic and the cells entrapped in them maintained their viability.
4.2.3 Electromagnetic Forces (Continuous, Pulsatile)
4.2.3.1 Electrical Forces in Tissue Engineering
All cells are known to have a voltage difference across their membranes called the membrane potential. This potential is a result of the ion concentration differences between the two sides of the membrane. For every ion, the potential difference generated can be calculated using the Nernst equation:
where EMF is the electromotive force (mV), R the ideal gas constant, T temperature in Kelvin, F is the Faraday constant, z charge of the ion, and [A]in and [A]out are concentrations inside and outside the cell membrane.
Equation 4.1 is applicable only when the membrane is in thermodynamic equilibrium. Due to the presence of active ion pumps on the cell membrane, there is never an equilibrium between the inside and the outside of the membrane, and therefore, the membrane potential cannot be calculated from the Nernst equation. In such a case, the resting potential of a cell can be calculated using the Goldman–Hodgkin–Katz equation:
where Pion is a permeability factor for each ion and the subscripts i and j are for the positive and negative charged species.
These equations take into account that cellular electromotive forces are generated by the ionic gradients across the membrane. Although all cells have a potential across their membranes, some cells are specialized in generating and conducting these electrical forces [13]. Most important examples of such cells are neurons, cells of the conducting system of the heart, and the cardiac and skeletal muscles. These cells, unlike other cells of the organism, use ion channels and cellular junctions actively not just to create a membrane potential but also to manipulate them to elicit action potentials.
Tissue engineering constructs are designed to mimic different physical, chemical, and biological aspects of a given tissue to generate a product that can meet the functional and structural demands of the original tissue to be assisted or substituted. From this point of view, electrical forces are used in tissue engineering applications in two ways: (a) to mimic the original (i.e., nervous or cardiac tissue), by generating a scaffold that can be used to transmit an externally applied or internally generated potential across its surface to align and orient cells and (b) to make a design so that even if the engineered tissue itself does not have the intrinsic electrical force generating capacity, the potential applied may lead to the changes that would guide the cells in the desired direction (i.e., differentiation).
Nanowires (NWs) are commonly used for these applications. For example, Long et al. prepared poly(3,4-ethylenedioxythiophene) (PEDOT) NWs over templates of track-etched poly(carbonate) membranes [14]. NWs with 190 nm, 95–100 nm, 35–40 nm, and 20–25 nm diameters were tested for their conductivity and were found to have different characteristics (critical, metallic, and insulating), in relation to their diameters. Carbon nanotubes are commonly used in conductive/semiconductive composites. Single-walled carbon nanotubes (swCNTs) behave as semiconductors or metals depending on their diameter, while multiwalled carbon nanotubes (mwCNTs) are always conductive. In a study by Sulong et al., mwCNTs were functionalized by carboxylation and octadecylation [15]. Results show that unfunctionalized carbon nanotubes are more suitable for electric applications because chemical functionalization of CNTs significantly decrease electrical conductivity. Among the polymers, polypyrrole, polyaniline, and poly(3,4-ethylenedioxythiophene) are the most commonly used conductive polymers [16].
Nerve injury, whether central or peripheral, is a catastrophic event. Most of the approaches to nerve injury today include surgical repair of the spinal cord or the peripheral nerves. Surgical repair options include end-to-end suturing and nerve grafts, with quite promising results, but these techniques also have their shortcomings [17]. Tissue-engineered nerve conduits are being increasingly used in their repair; the choice of the right material is very important and their biochemical functionalization or topographic modifications are frequently used in material improvement [18].
From the materials point of view, several polymers and nanocomposites have been studied for their electrical conductivity or suitability for conversion into conductive materials when they are normally nonconducting. Using electrical forces to direct, align, or orient nerve cells to achieve neuronal function was the subject of many recent studies. Bechara et al. extruded poly(caprolactone) (PCL) through nanoporous membranes to obtain NWs, which were coated with the conductive polymer poly(pyrrole) (PPy) [19]. C17.2 murine neural stem cells (NSCs) were cultured on these NWs, and physiological levels of electrical stimulation was applied. Results showed that PPy coating decreased the resistivity of PCL NW. Quantitative microscopic analysis of NSC using calcein-AM staining demonstrated an increase of more than twofold in proliferation using PPy–NW surfaces instead of only NW. Schmidt et al. synthesized oxidized PPy films and laminated them with poly(lactic-co-gylcolic acid) (PLGA) to increase their mechanical stability [20]. Rat PC-12 cells and sciatic nerve explants from 16-day-old chick embryos were cultured on these substrates. As judged by the neurite lengths of cells stimulated with 100 mV for 2 hours, the application of electrical stimulus enhanced PC-12 cell differentiation. In another study with PPy films, surfaces were bathed with a fibronectin solution to increase cell adhesion, and potential was applied [21]. It was observed that electrical force enhanced neurite outgrowth on the fibronection-coated surfaces.
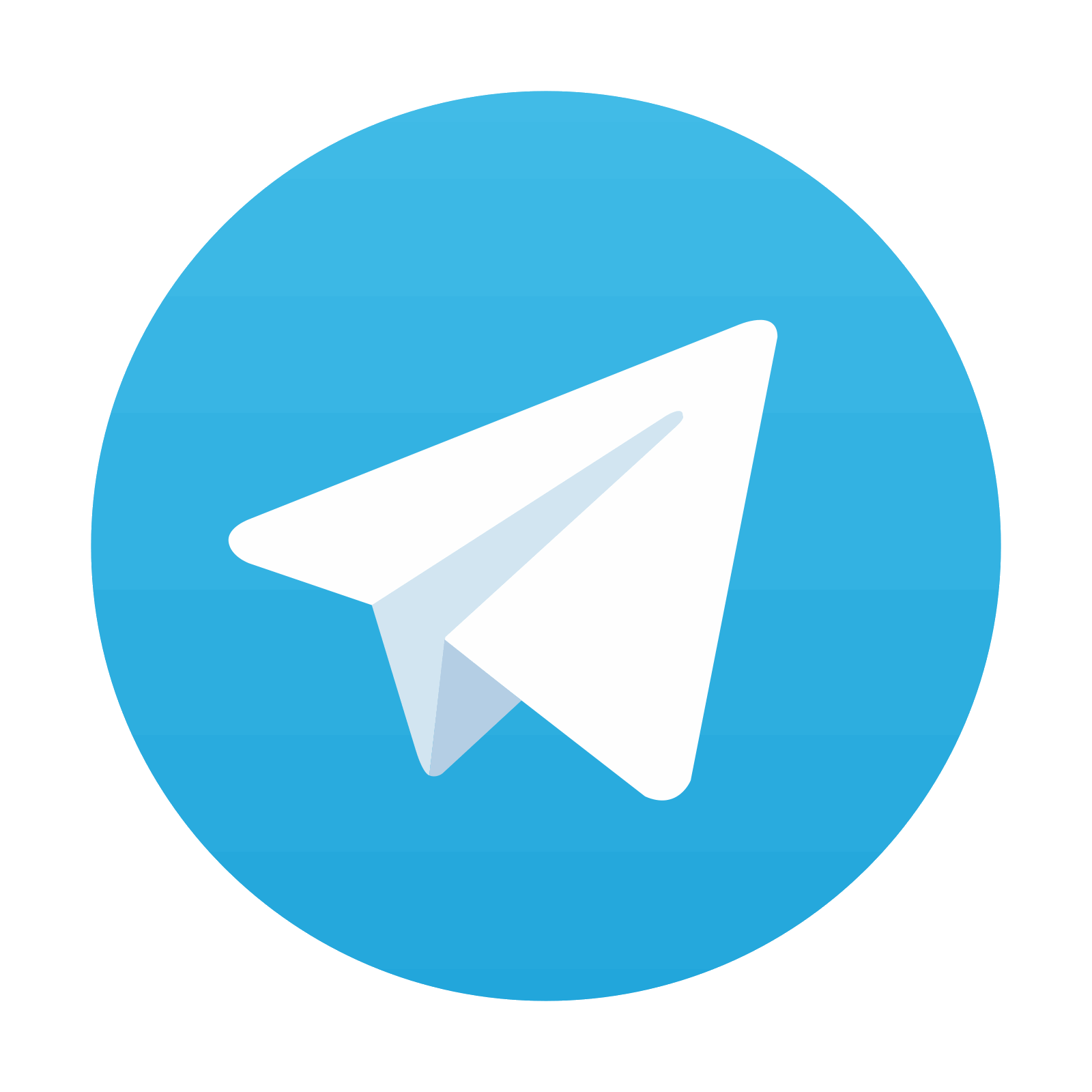
Stay updated, free articles. Join our Telegram channel
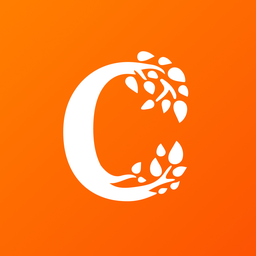
Full access? Get Clinical Tree
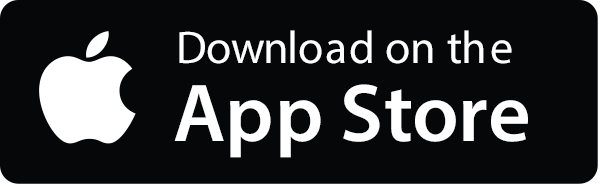
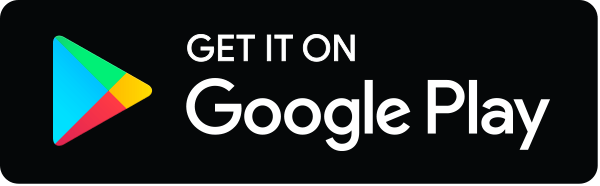