The Role of Mechanical Cues in Regulating Cellular Activities and Guiding Tissue Development
Division of Biomedical Engineering, Department of Mechanical and Automation Engineering, The Chinese University of Hong Kong, Shatin, New Territories, Hong Kong
3.1 Introduction
Mechanical cues impact virtually every organ system in our bodies including musculoskeletal, cardiovascular, pulmonary, dental, and neural tissues. These mechanical cues can be in the form of compression, tension, torsion, and fluid shear. An increasing amount of research is being devoted to understanding the effect of mechanical signals on cell behavior and tissue morphogenesis. Significant progress has been made in elucidating the molecular mechanisms by which individual cells sense these mechanical signals and translate them into cascading cellular signaling events and ultimately, gene expression, a process that is known as mechanotransduction. In this chapter, we will discuss the major discoveries in mechanotransduction and the role of mechanical signals in guiding tissue development in various physiological systems.
3.2 Mechanotransduction
Cells possess a plethora of mechanosensitive entities on their surface such as ion channels, extracellular matrix (ECM) binding receptors, and specialized cell membrane structures [1–3]. The downstream signaling cascades triggered by mechanical sensing lead to changes in gene expression in the cell nuclei [4,5]. Because mechanical signals propagate through long distances via physical cellular structures in the cells, the speed of the mechanically induced signaling is predicted to be significantly faster compared with signaling mediated by diffusion of soluble factors [6,7]. Along the propagation path of mechanical signals from cell surface to intranuclear genetic machinery, there are two critical steps of mechanotransduction; firstly, signal transfer from extracellular space to intracellular cytoplasm and secondly, from cytoplasm to intranuclear genetic machinery.
3.2.1 Mechanotransduction from Extracellular Matrix to Cytoplasmic Structures
Cells possess a number of membrane-based sensory structures that probe and detect external forces (also reviewed by DuFort, Paszek, and Weaver) [8]. This force sensing, also termed mechanosensing, is generally mediated by conformational changes in force-sensitive cellular molecules or structures, such as cadherin complexes in cell–cell adhesions, mechanosensitive ion channels, G protein-coupled receptors, kinase receptors, and integrins [3,9–11]. The detected mechanical cues generate specific signals that are propagated through cytoplasmic molecular structures leading to the activation of mechanosensitive intracellular signaling pathways. For instance, integrins are capable of aggregating and binding to extracellular molecules such as fibronectin and vitronectin to form focal adhesion (FA) complexes [12]. External mechanical stains can be transmitted through these integrin-mediated adhesions to modulate intracellular molecules such as focal adhesion kinase (FAK) [13] (Figure 3.1). These molecular changes initiate a cascade of signaling events including the activation of Rho-family GTPases (enzymes that hydrolyze guanosine triphosphate (GTP)), such as RhoA, which promotes actin restructuring, induces protein phosphorylation, and regulates gene expression by influencing transcription factors [14]. Through received external mechanical signals, integrins also impact the mitogen-activated protein kinase and extracellular signal-regulated kinase (MAPK–ERK) pathway, which has been implicated in cell proliferation and differentiation [5,15].

3.2.2 Mechanotransduction by Cell–ECM Adhesions
One class of the most-studied mechanosensory complexes is the FAs developed at the binding sites of integrins to ECM molecules [16]. The FA complexes consist of not only integrins but also an array of cytoplasmic adapter and signaling proteins, including vinculin and talin, which connect the intracellular domain of integrins to actomyosin cytoskeleton [17] (Figure 3.1). These cross membrane molecular complexes serve as the link between the ECM structures and the intracellular cytoskeleton and allow transmission of mechanical signals from the outside into the cellular space in response to physical forces. The FA complexes are dynamic rather than static, and external forces can lead to conformational changes in proteins within the FAs, including the transmembrane integrin and adapter proteins such as talin, effectively converting mechanical signals into biochemical ones [18,19]. The effects of these force-induced protein conformational changes on protein–protein interactions depend on the specific molecules involved. For example, when integrins bind to the ECM molecule fibronectin, the conformational change in integrins generally stabilizes the binding. In contrast, the force-induced conformational change in talin helps unravel the molecule, leading to the exposure of otherwise inaccessible vinculin-binding sites [17,20]. Talin–vinculin binding in response to mechanical signals promotes the clustering of integrins and the aggregation of adhesion plaque proteins, which enhances the activation of signal transduction molecules at the cytoplasmic side of the FA complexes [5].
3.2.3 Mechanotransduction by Cell–Cell Adhesions
Cell–cell adhesions, including adherens junctions (AJs), tight junctions, and gap junctions, also play an important role in mechanotransduction [21–23] (also reviewed by Chen, Tan, and Tien) [24]. Among these different cell–cell adhesions, the AJs have received the most research attention for their capability to directly transmit external mechanical signals into intracellular actomyosin activities. The AJs are mediated by the homotypic binding of extracellular domains of cadherins, a family of transmembrane Ca2+-dependent adhesion molecules, expressed on the surfaces of the neighboring cells [25]. On formation of AJs, scaffolding proteins are recruited by the cytoplasmic domains of cadherins and help anchor the actin cytoskeleton. FAs and AJs share many similar attributes. Firstly, both are formed by transmembrane receptors that are capable of transmitting external mechanical signals to intracellular actin cytoskeleton. Furthermore, in both FAs and AJs, the transmembrane receptors form clusters and recruit, with their intracellular domains, signaling, and structural molecules that can interact with either the actin cytoskeleton or other relevant molecules. In addition, many of these recruited intracellular proteins, some of which are shared by these two types of adhesions such as vinculin, can undergo conformational changes in response to perceived mechanical cues and thereby convert mechanical signals into biochemical ones, which ultimately influence transcriptional activities in the nuclei [26].
3.2.4 Intracellular Molecules
On ligand binding, integrins form clusters and initiate recruitment of actin filaments to the integrin cytoplasmic domain. The actin recruitment is facilitated by the direct or indirect binding of a number of structural and signaling proteins including talin, vinculin, α-actinin, and filamin to the integrin cytoplasmic domain. Talin, vinculin, and FAK (or PTK2, a cytosolic tyrosine kinase) play a key role in the mechanical integrity of FA complexes and are correlated with the strength of the adhesions [27]. Talin is a large dimeric protein that attaches integrins to the actin cytoskeleton and is indispensable to the structural integrity of FAs [28]. Furthermore, ligand-binding activity of integrins and the recruitment of signaling proteins like FAK are also regulated by talin [29]. Vinculin does not bind integrins directly, but facilitates FA formation indirectly by connecting talin and α-actinin to the actin cytoskeleton and recruiting additional proteins such as paxillin and vinexin [30]. The amount of vinculin recruited to FAs correlates with the extent of local tension [15,31]. FAK is one of the molecules in FAs that respond to mechanical signals the quickest. Increasing cytoskeletal tension or application of extracellular forces can enhance FAK phosphorylation, leading to increased cell proliferation [32]. Force-induced FAK tyrosine phosphorylation also plays an important role in cell migration in response to mechanical cues such as fluid shear [33]. β-Catenin, which binds to the cytoplasmic domain of cadherins, is a structural element in AJs which links cadherins to the actin cytoskeleton with the help of other adapter proteins [34]. β-Catenin can dissociate from AJs on phosphorylation and translocates to the nuclei to interact with transcription factors leading to modulation of gene expressions [35,36].
3.2.5 Adhesion‑Mediated Signaling Pathways
Cytoplasmic signaling from integrin-mediated adhesion is typically characterized by two phases. Early adhesions lead to activation of pathways, such as those involving Rac and Cdc42 that stimulate actin polymerization and membrane protrusion, whereas established adhesions activate pathways such as the RhoA signaling pathway that promote cytoskeletal contractility and transmission of tension to the FAs [37]. Cdc42, Rac, and RhoA all belong to the Rho family of GTPases. Cdc42 and Rac regulate the development of filopodia and lamellipodia during cell adhesion and spreading on ECM, respectively [38]. The tensile forces that cells exert against their adhesions have been shown to positively correlate with externally applied forces or cell spreading [39,40]. RhoA, through its effector Rho kinase (ROCK), plays a key role in the formation of stress fibers and generation of cytoskeletal tension in adherent cells [41]. ROCK promotes phosphorylation of myosin II regulatory light chain (MLC) to increase myosin II contractility and intracellular tension, leading to cell proliferation [42,43].
3.3 Mechanotransduction from Cytoplasm to Nucleus
The mechanotransduction in cytoplasm and nucleus has been comprehensively reviewed previously [44]. Recent studies identified a specialized structure, known as the linker of nucleoskeleton and cytoskeleton (LINC) complex, which helps anchor cytoskeletal structures such as actin filaments to the nuclear membrane. The LINC complex contains adapter molecules such as nesprins, SUN, and lamin proteins [45] (Figure 3.1). Nesprins isoforms (nesprin 1 and nesprin 2) are rod-like nuclear membrane proteins that connect actin filaments to SUN1 located on the inner nuclear membrane with their amino and carboxyl terminals, respectively [46]. SUN1 connects this anchoring structure to lamin A on internal nuclear scaffold, as well as nuclear pore complexes, and therefore may be involved in mechanical regulation of nuclear pores [47].
Lamins A, B, and C are structure proteins that form a molecular network on the nucleoplasmic surface of the inner nuclear membrane. Lamins are also components of internal nuclear scaffold and play a vital role in the control of nuclear organization and regulation of gene expressions [48]. Lamin A and lamin C (A-type) have been shown to be more closely involved in mechanotransduction compared with lamin B (B-type) [49]. It is postulated that lamins connect to the genetic machinery both directly and indirectly by partnering with other nuclear proteins such as emerin [48,50,51]. Emerin binds to the LINC complex through nesprins and lamins, and together with barrier-to-autointegration factor (BAF), connect lamins to polymerized nuclear actin, resulting in enhanced actin polymerization [52,53]. In addition, actin and myosin are also involved in modulating nuclear structure and nuclear functions, such as chromosome movements and transcription [54,55]. Therefore, it is likely that actomyosin interactions also facilitate force propagation and transduction in the nucleus.
The structure and organization of the genome itself is organized into loops and is influenced by attachment to nuclear matrix attachment regions (MARs). External factors and induced cell differentiation can change the genome organization and nuclear matrix [56]. Many nuclear proteins could have a dual role not only in the regulation of DNA transcription but also in the contribution to the mechanical properties of nucleoskeleton. For instance, Runx2, a critical transcription factor required during osteogenesis, also seems to function as a nuclear structural protein and links different regions of chromosomes, thereby potentially assisting in the regulation of combinatorial gene transcription [57,58]. Therefore, mechanical signals propagating through cytoskeleton and then the LINC complex can directly impact crucial DNA regulatory enzymes and binding factors via the interconnected nuclear scaffold.
3.4 Role of Mechanical Cues in Developmental Biology
Since the advent of modern molecular biology, an increasing emphasis in developmental biology research has been placed on the understanding of cellular events based on molecular signaling cascades, with little consideration for the impact of physical cues from the larger mechanical context at tissue and organ level, in which the cells are residing. As discussed in the previous sections, this mechanical context is the physical continuum from tissue and organ level to cell level as a collective outcome of cell–cell adhesions, cell–ECM interactions, and linking of these two adhesions to intracellular cytoskeletal and nucleoskeletal structures in tandem. Cell behavior and therefore tissue morphogenesis are strongly influenced by the mechanical signals from the environment, including mechanical information in the form of biophysical stimuli such as compression, tension, fluid shear, and so on.
The effect of mechanical signals on tissue and organ development, especially on skeletogenesis, has clearly been demonstrated in established animal models. For example, immobilization of chick embryos using neuromuscular blocking agents abrogates muscle dynamic contractions and lowers muscle forces. This induced paralysis had a dramatic effect on animal bone development and resulted in abnormal curvature of the mandible, neck, and spine, and retarded growth of clavicle, femur, tibia, and humerus [59]. Hind limb muscular atrophy, which was induced by excising the neural tube, resulted in significantly short femur and tibiotarsus compared with controls. The bulk bone stiffness and bending strength of tibiotarsi of immobilized chick embryos were also significantly reduced due to the lack of muscle contraction [60]. The dramatic effects of muscle contraction-induced mechanical stimulation on mammalian bone development were also clearly demonstrated in genetically modified mice with absent or noncontractile muscles [61–63]. In mice with double knockouts of Myf5 and MyoD, the normal development of the anatomical structure and geometry in cervical vertebrae, spine, mandible, palate, clavicle, and sternum was substantially compromised [61]. Numerous studies have also shown that muscle contraction is critical to joint cavitation. Cavitation of the knee, ankle, hip, and toe joints was minimal or absent in animal treated with drugs that blocked muscle contraction. When muscle force was eliminated by the surgical excision, effects on joint cavitation similar to those in the drug-treated animals were observed. Furthermore, immobilization of embryos after the formation of joint cavity resulted in the loss of the cavity, and wider joint cavities in the hip, shoulder, knee, hands, and feet developed after muscle force was pharmacologically restored [31,64]. It was found that changes in ECM molecules were responsible for the morphological abnormalities due to immobilization [65]. Studies showed altered patterns of tenascin-C, extracellular-regulated kinase, fibroblast growth factor (FGF)-2, and collagen-XII in immobilized limbs and joints [66,67]. The removal of muscle contractions also affected the structures associated with the joint. In immobilized embryos, even though the presence of meniscus was evident at day 8, they started to degenerate by day 10 and disappeared by day 11 or 12, and the plantar tarsal sesamoid completely failed to form [68]. This indicated that mechanical loading is essential for sesamoid formation and late stages of meniscal development and maintenance [68].
Dynamic patterns of biophysical stimuli in the joint colocalize with the development of patella and articular cartilages. Changes in joint shape may have been due to changes in the tissue properties of immobilized joints, since immobilization seems to differentially affect the mechanical properties, glycosaminoglycan (GAG) (proteoglycan), and total collagen content in different areas of joint epiphyses.
The key role of mechanical forces played in bone and joint formation, as demonstrated by developmental models with abnormal skeletal muscle, has been thoroughly summarized and reviewed by Nowlan et al. [69] (Figure 3.2). However, relatively little is known about how biophysical stimuli are translated into gene regulation via molecular mechanisms. Most of the previous studies of mechanotransduction signaling were conducted in the classic two-dimensional (2-D) monolayer culture, which may not fully reflect the complexity in the three-dimensional (3-D) multicellular tissue environment in which cells reside. Refinements on the animal models, such as more sophisticated genetic manipulation, generation of more realistic in vitro
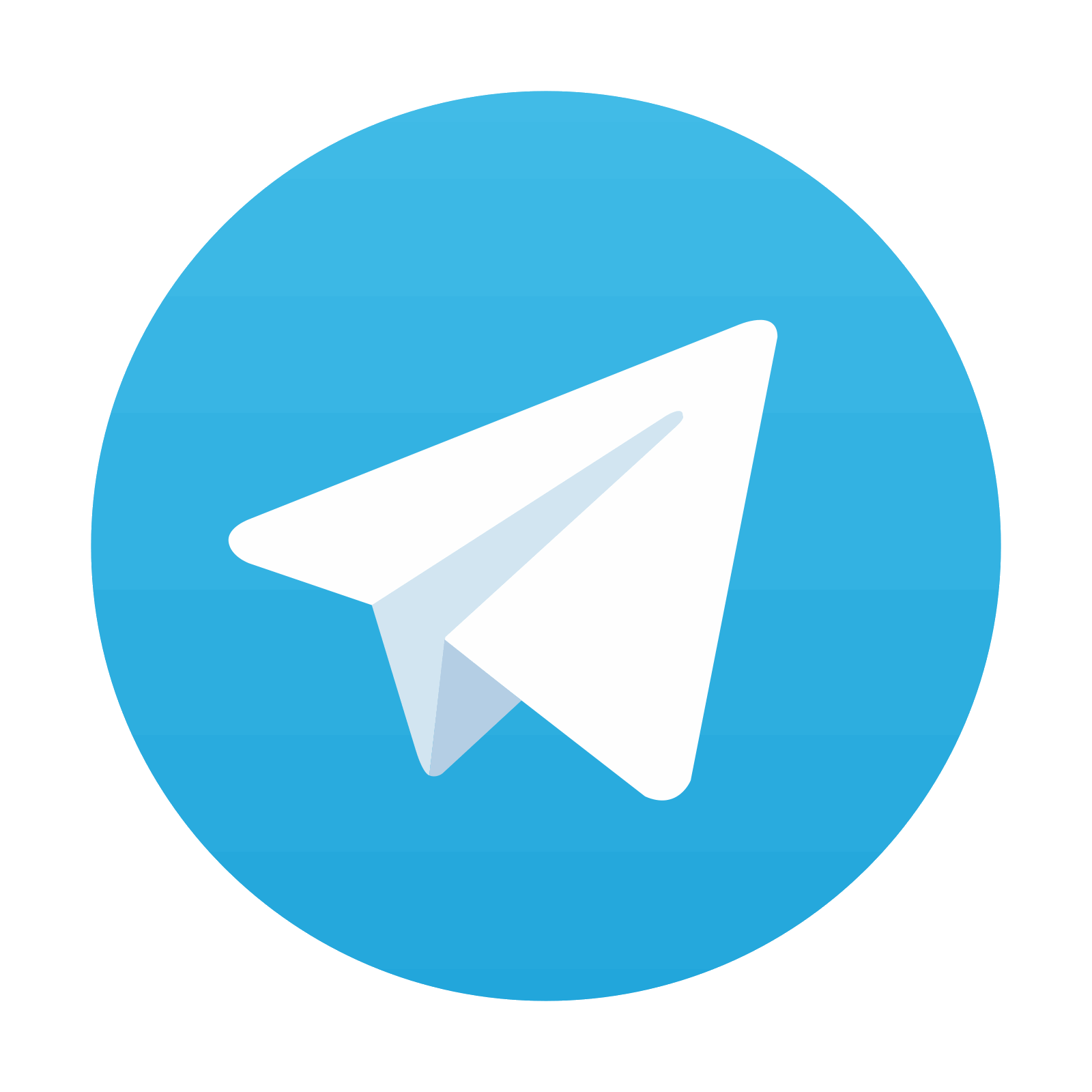
Stay updated, free articles. Join our Telegram channel
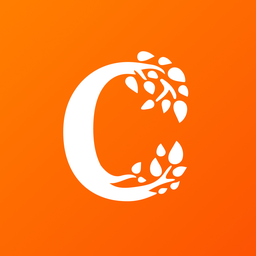
Full access? Get Clinical Tree
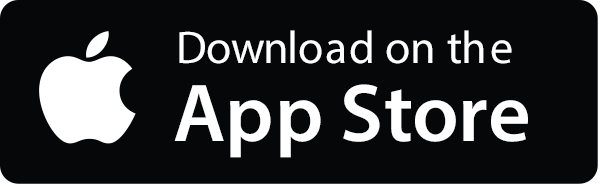
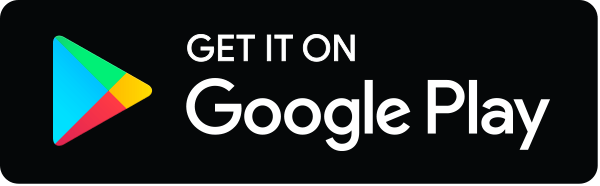