CHAPTER 24 Natalie N. Whitfield1, Raquel M. Martinez2, and Donna M. Wolk2 1 University of Arizona Medical Center, Tucson, AZ, USA 2 Geisinger Health Systems, Danville, PA, USA In the first edition of this text, Wolk and Persing asked the question, “What will the future hold in terms of laboratory diagnosis of infectious disease?” [264]. In 2002, they predicted that clinical microbiologists would face ample opportunities to change the face of their practice, and predictions for change included the following: The preceding list of 20 items, predicted in 2002, creates a clear and singular description of clinical microbiology diagnostic practice today. Since the first edition of this book, diagnostic capabilities in clinical microbiology have grown exponentially, in part due to the impact of new molecular and proteomic tools to detect human pathogens. In the first edition of this book, the routine integration of real-time PCR and robotic instrumentation for PCR, reverse transcription (RT) PCR and nucleic acid extraction was predicted [264]. Most of the technology previously reviewed is now either commonplace or has come and already gone with the rush of new technology in clinical microbiology. The technologies cited in the first edition have evolved by several generations in many cases, with new software and engineered improvements. Real-time PCR, molecular beacon probes, DNA sequencing, and robotic nucleic acid (NA) extraction, have become routine, and best practices for these methods have made their way into guidelines from the Clinical and Laboratory Standards Institute (CLSI) and College of American Pathologists (CAP) checklists. Solid microarrays have not yet become entrenched, but their liquid version counterpart, Luminex-based multiplex assays, and multiplex assays with integrated detection systems that followed (Nanosphere, GenMark, and BioFire) have revolutionized the way we perform virology alongside bacteriology as routine components of diagnostic testing for respiratory pathogens. New technology will continue to rapidly influence the diagnostic sciences. This chapter will focus on recently introduced or novel methods and advances that have changed or are poised to change the landscape of clinical microbiology. Trends in identification of human pathogens, commonly encountered in diagnostic settings, will also be discussed. Of note, this chapter focuses primarily on technology that is US Food and Drug Administration (FDA) reviewed, approved, or being targeted toward FDA clinical trials; no trademarks or similar notations will be used in this chapter, please consult with the manufacturers to obtain this information. In this review, the reader will learn how new technological advances provide us tools with which we can attempt to improve patient care and disease management. In addition, we will detail examples of how clinical microbiologists can document both benefits and limitations of new technology and related clinical practices. The benefits of technology generally include the ability to provide fast results, increased sensitivity and specificity, greater versatility, and more accurate results that are not necessarily dependent on isolation of pathogens in culture. An additional benefit is the ability to obtain multiple answers from one test process; for example, the result may document the presence of multiple pathogens or identify the pathogen and common antimicrobial resistance markers. Their limitations generally include: (i) higher costs; (ii) inability to detect emerging genetic or proteomic sequences that may not be targeted by current primer or probe combinations; (iii) inability to detect sequence variants not yet placed into genomic databases; and (iv) the inability to access cultivated microbes for susceptibility testing or genotyping. Over the past 12 years, clinical microbiology strategies transitioned from a primary “culture all pathogens” approach to a combination of culture and “amplify multiple genetic targets” approach. The excitement surrounding rapid development and diversity of new technology is tempered by current healthcare expectations to describe clinical and financial utility of laboratory testing prior to, or quickly after implementation. As first noted in 2002, Wolk stated; “We have opportunities to shape the future of our clinical practice. Progress must be tempered with common sense so that optimal patient benefit is achieved and documented [264].” Although the evidence-based laboratory intervention literature is currently sparse, there is hope that the merits of recent improvements will be documented to increase the cost-benefit of such laboratory testing [234,235]. In general, purified NA is necessary as the primary template for most amplification technologies and may be performed as a preliminary step for diagnostic assays. Starting from a clinical sample, NA extraction can be performed by either manual or automated methods (Table 24.1). Determining which method to use is a critical step in successful NA purification and use in downstream amplification assays. The extraction methods that may work for one pathogen and specimen type may not necessarily be effective for other specimen types and pathogens. In addition, structural characteristics of microorganisms and specimen inhibiting substances can affect NA extraction. Table 24.1 Common automated nucleic acid extraction systems * Automated instrumentation with extraction module and assay preparation. Not available as a stand-alone extractor. Manual methods are time-consuming and are generally less reproducible than automated methods, and they are moving towards extinction in clinical laboratories where automation is overtaking manual methods. With today’s assays moving from single-target assays to those with multiple target amplification, the extraction requirements are more important than ever before, requiring optimized upstream NA extractions that produce high yield and high purity extract [19]. Automated linked or integrated extraction systems are becoming more common and practical for use in clinical laboratories. Linked automated extraction systems incorporate a modular approach to extraction, amplification, and target detection, typically two or three different instruments are linked by software that recognizes and tracks samples via barcodes as they move, either robotically or manually through the molecular testing process. Linked extractors are primarily intended for use with only certain downstream detection systems, typically sold by the same manufacturer or a corporate partner of the manufacturer. Thus, while adding ease and additional throughput to molecular laboratory processes, a linked system typically locks the user into purchasing only the testing reagents that are designed for use with that one system, limiting flexibility in reagent selection. In contrast, integrated systems are those in which the processing cartridge incorporates all phases of testing, namely, extraction, amplification, and detection. Advantages of these systems include the speed of results and common Clinical Laboratory Improvement Amendments (CLIA) classification of moderate complexity. Limitations include higher costs and smaller throughput than the larger linked systems, which commonly base testing on 48–96 tests per run. Linked and integrated systems provide several benefits over manual extraction methods, which are losing favor in many busy multitasking molecular laboratories feeling the effects of workforce shortages and limited resources. Moreover, automated NA extraction systems tend to yield more reproducible NA recovery and reduce the risk for contamination of other samples and the work environment [94]. The robotic components of linked or integrated automated extraction instruments ensure greater workload efficiency with very minimal manipulation or attention [117,258]. Many offer inventory control and full process documentation to support best practices in clinical laboratories. One example of a new concept, the linked automated extraction platform, is the Qiagen QIAsymphony SP system (Qiagen, Germantown, MD). The QIASymphony (Figure 24.1a) is designed to couple with the QIAsymphony AS (assay setup) module (Figure 24.1b) for automated PCR assay preparation and the Qiagen Rotor-Gene Q real-time PCR instrument for target amplification (Figure 24.1c). Integrated extraction systems, such as the QIAsymphony, typically provide prefilled reagent cartridges, bar-coding of samples and reagents, and touch-screen operation. Many robotic NA extraction systems allow loading of 1–96 samples per run, and some can perform different purification procedures within the same run of 96 samples. Figure 24.1 (a) Qiagen’s QIAsymphony SP instrument and integrated extraction system, is designed to work in conjunction with (b) the QIAsymphony AS (assay setup) module, and (c) the Qiagen Rotor-Gene Q, real-time PCR instrument. (© Qiagen, all rights reserved.) Other examples of linked extraction platforms include the Roche COBAS AmpliPrep/COBAS TaqMan System (Roche Molecular, Indianapolis, IN), and the Abbott m2000sp (Abbott, Des Plaines, IL). The COBAS AmpliPrep platform (Figure 24.2a) isolates RNA or DNA with silica-coated glass magnetic beads and is linked with the COBAS TaqMan Analyzer (Figure 24.2b) or smaller COBAS TaqMan 48 Analyzer for real-time PCR and amplicon detection. Process integration occurs via the Roche AMPLILINK software. The system has a throughput of 168 samples in an 8-h shift and can load up to four different tests at once. Figure 24.2 (a) The Roche COBAS system is comprised of the COBAS AmpliPrep instrument and (b) the COBAS TaqMan instrument. The two components are depicted here in “docked” mode, but can also be undocked and placed in separate laboratory areas. (Courtesy of Roche Diagnostics Corporation.) The Abbott m2000sp (Abbott, Des Plaines, IL) system (Figure 24.3a) is used for sample preparation and is integrated with their real-time PCR downstream applications performed on the Abbott m2000rt: RealTime PCR platform (Figure 24.3b). Throughput options range from 24 to 96 samples and the system has an advantage of a partially open mode, which can allow the users to alter the protocols for various sample types and input volumes. In addition, the m2000sp can be used with reagents from a corporate affiliation with Intelligent MDx (Cambridge, MA). Please refer to other chapters in this text for additional information. Figure 24.3 (a) Abbott’s m2000 sp system for sample preparation is linked with (b) m2000rt for real-time amplification and detection to form a multicomponent system for qualitative and quantitative PCR and RT-PCR. (Courtesy of Abbott.) Other emerging linked technologies include the Sentosa system (Vela Diagnostics, Fairfield, NJ), in which an Eppendorf robotic pipettor was adapted to become the Sentosa SX101, and combined with a Qiagen Rotor-GeneQ real-time cycler and options for an Ion Torrent Sequencer, adapted and branded as the Sentosa SQ301 (all sold and supported for research use only (RUO) by Vela Diagnostics). Vela currently offers a CE-IVD test, Sentosa SA Direct MRSA/SA PCR, for qualitative detection of Staphylococcus aureus (SA) and methicillin-resistant S. aureus (MRSA) from nasal swabs. They also offer RUO PCR-based surveillance testing for the identification of the mosquito-borne Chikungunya virus. Despite the advantages of automated and integrated systems, there are circumstances when manual methods may be preferred or used in combination with automated methods. For example, manual methods may offer higher DNA yield or purity, or may be useful when microbial cell walls or inhibitors in specimen matrices become problematic. Manual extraction kits are usually less expensive, but do require several manipulations, which may offset the savings in reagent costs. Lastly, automated linked and integrated extraction instrumentation can require the use of large footprints of space, a valuable commodity in many laboratories. According to predictions, real-time PCR is arguably the molecular method with the most profound impact on the clinical laboratory in the past two decades. Since its emergence in clinical laboratories in the late 1990s, real-time PCR integrated into diagnostic practice relatively quickly, serving as a rapid method to identify or quantify many common pathogens [25,59,257,260]. PCR methods continue to evolve and redefine the optimal diagnostic strategy in clinical microbiology laboratories. With the development of even simpler and more rapid methods, multi-target chemistries, smaller instrument footprints, integrated extraction, and amplification with minimal manipulation [59,60], real-time PCR methods are steadily supporting our ability to improve patient care. Such benefits currently decrease the time to result, the hands-on time demand and requirements for qualified molecular technologists to perform the testing. It is not surprising that molecular pathogen identification is strikingly more common than it was a decade ago. There are now several assays and instruments available, which are classified as “moderate complexity” by CLIA, making them amenable to testing by most laboratory staff, for 24/7 testing, which can be used in small community hospital laboratories and large reference laboratories alike [25,59]. With the advent of completely integrated systems, the ability to apply such technologies to direct point-of-care testing and bedside identification is promising. Currently, two main types of diagnostic real-time PCR assays are used for the detection of pathogens: (i) multi-step real-time PCR assays that require off-platform specimen processing, extraction of NA from the specimen, PCR assay set-up, and amplification/detection; and (ii) integrated assays that incorporate the three steps into the subsequent amplification/detection step. Examples of the latter type, in order of appearance to the US market, include Cepheid’s GeneXpert system, Becton Dickinson’s BD Max system, BioFire Technology’s FilmArray and Focus Diagnostic’s Simplexa Assays on the Integrated Cycler. The benefits of the integrated real-time PCR assays are decreased hands-on time, no manipulation of the extracted nucleic acids, which decreases the risk of contaminating the work area, and in most cases decreased reaction time and overall turnaround time. Since there are hundreds of real-time PCR examples, all cannot be reviewed here and we refer readers to recent extensive reviews of methods [78,257,260]. Likewise, it is not possible to review the entire breadth and depth of laboratory-developed tests (LDTs). We will review examples of common or emerging technology with impact to clinical microbiology laboratories. Focus Diagnostic (Cypress, CA) markets the eight-well Simplexa Direct Amplification Disc assays (Figure 24.4a) that are fully integrated and do not require any upstream NA extraction steps, thus decreasing turn-around time and enabling performance of molecular testing in places where engineered molecular laboratory space is not available. Simplexa Direct assays include influenza A/B and respiratory syncytial virus, herpes simplex viruses 1 and 2, and Group A Streptococcus. Simplexa assays are performed on the four-channel 3M Integrated Cycler (Figure 24.4b), which allows processing of multiple pathogen targets simultaneously. The growing test menu of molecular assays includes detection of Clostridium difficile, Bordetella pertussis/parapertussis, Chlamydia (Chlamydophila) pneumoniae, Group A Streptococcus, Mycoplasma pneumoniae, and Borrelia spp.directly from patient specimens [57,127,248]. Figure 24.4 (a) The Focus Diagnostics eight-well Simplexa Direct Amplification Disc combines with (b) the 3M Integrated Cycler for direct amplification and detection without the need for nucleic acid extraction. (Courtesy of Focus Diagnostics) For assays in which NA extraction is required prior to sample loading, amplification and detection of up to 96 samples can occur on one Simplexa disc, with results available in about an hour after loading the instrument. The assays and instrument support software programs for DNA and RNA, qualitative and quantitative PCR reactions, and a small instrument footprint. Assays require small sample volumes and multiple assays can be performed on the same disc. The menu of PCR and RT-PCR Simplexa assays is extensive. IntelligentMDx (Cambridge, MA) uses a unique proprietary bioinformatics process to provide analysis and verification of genetic sequences, thus producing assays with the potential to quickly adapt to genetic change. The IntelligentMDx bioinformatics process, NGENix, was specifically developed to address complex and evolving infectious targets. The NGENix system and processes may enable a better understanding of NA interactions and enhance the development of assays for any molecular target. Accelerated production of assays occurs by preselection of metrics, algorithms, and rules necessary to build robust, reproducible molecular assays for the detection of pathogens. The Intelligent MDx process is the first to use a systems approach and multifactorial computational capability that enables in silico assay design for primers, probes and amplification conditions. Built into this process, is the integration of input from expert end-users to preset the clinical indications and specifications for an assay. In theory, the assay should be robust and easily adapted to emerging genetic sequences as pathogens evolve. Real-time PCR assays are designed with similar reaction conditions so that they can be multiplexed, performed in parallel, or performed separately, allowing clinical laboratories to optimize throughput and workflow. There are three current IntelligentMDx assays commercially available in the United States. The C. difficile assay for the Abbott m2000 is a PCR assay for the qualitative detection of conserved regions within toxin A and toxin B genes of the C. difficile genome in human symptomatic patients. The assay detects toxigenic C. difficile, including NAP1/027 hypervirulent strain and toxin B-variant type strains. In addition, the VanR assay detects vanA and vanB vancomycin resistance genes directly from human perirectal or rectal swabs or stool samples from patients at risk for vancomycin-resistant Enterococcus (VRE) colonization. More recently, the Flu A/B and RSV assay was approved for testing nasopharyngeal swabs and includes detection of the emerging H3N2 variant strains. All IntelligentMDx assays are intended for use on the Abbott m2000 RealTime system, a high throughput system ranging from 22 to 96 samples per batch (Figure 24.3a,b). Digital PCR is noted to have potential for highly accurate and reproducible quantitation of viruses and other genetic targets [16,50,83,88,184,241]. Droplet Digital PCR (ddPCR) from Bio-Rad (Hercules, CA) is a technique based on partitioning of many PCR reactions in water-oil emulsion droplet technology. Bio-Rad’s QX100 system fractionates each sample into 20,000 individual nanoliter-sized droplets (Figure 24.5), and PCR amplification of the template molecules occurs in each individual droplet. PCR-positive and PCR-negative droplets from every sample are then counted to provide absolute target quantification in digital form. The ddPCR technology uses reagents and workflows similar to those used for most standard TaqMan probe-based real-time PCR assays. The massive sample partitioning is a key aspect of the ddPCR technique. Digital PCR has been successfully used for quantitation of HIV and cytomegalovirus, as well as qualitative analysis of pathogens such as S. aureus and Mycobacterium tuberculosis [88,92,116,185]. Figure 24.5 Bio-Rad’s QX100 Droplet Digital PCR system (ddPCR) is a novel way to accomplish quantitative PCR. (Courtesy of Bio-Rad Laboratories, Inc.) The Cepheid GeneXpert (Cepheid; Sunnyvale, CA) is a completely integrated single-use cartridge real-time PCR instrument for direct detection of pathogens from clinical specimens. Specimen processing and decontamination occurs along with DNA extraction, amplification, and detection of the genetic target all within the same cartridge. A result is rapid, within 2 h, for all assays and requires minimal hands-on time. The system is composed of the GeneXpert instrument, with throughput designed to range from 1 to 96 samples simultaneously (Figure 24.6a and 24.6b, respectively) and a computer containing the required assay software to interpret the results. The various cartridges all incorporate Bacillus globigii spores, which serve as internal controls for extraction and amplification, making the test technically effortless with little manipulation by the operator. Currently, Cepheid offers assay cartridges for several important pathogens (references are listed for the first published method comparisons of the FDA-approved product found using search terms Xpert or GeneXpert and the pathogen and/or the publications from multisite trials), including S. aureus [178,263], MRSA [202,262], C. difficile [102,172], VRE [21], Group B Streptococcus [74], enterovirus [120,167], Chlamydia trachomatis/Neisseria gonorrhoeae [76], and M. tuberculosis [18,90]; the latter assay has been associated with a recent eruption of publications, with more than 156 publications in 3.5 years. Figure 24.6 Cepheid GeneXpert systems range in throughput, from (a) one-cartridge to (b) a 96-cartridge instrument. (Courtesy of Cepheid) The GeneXpert instrument is a random access instrument with rapid testing capabilities. Each individual reaction module can detect up to six genetic targets in a single cycler. Another advantage for pathogen detection is its multiplex capabilities, detecting the pathogen as well as some antibiotic resistance genes. Strain typing capabilities are also available, as in the case of presumptive identification of C. difficile NAP1/B1 strains [8,172]; yet there is no consensus for the utility of reporting the presumptive strain type. Methicillin resistance of S. aureus [202,215,236,261–263], vancomycin resistance of enterococci [21,46,75,144,272], and rifampin resistance of M. tuberculosis are discriminated by the system [84,95,104,152,158,159,273], but as with any laboratory method, vigilance is required because antibiotic resistance genes can mutate quickly and exceptions can occur [17,26,37,229]. Because NA extraction is not required with the latest assays from Focus (Cypress, CA), the Simplexa Direct assays also bear mentioning in the integrated PCR section (Figure 24.4a,b). Due to the lack of an extraction step, the assay costs are relatively low and ease of use is high; however, in some cases there may be some loss in sensitivity when compared to other molecular methods for assays that include extraction steps for influenza, RSV, and C. difficile [43,96,125,126,166,226,266]. Please see other chapters in this text for additional information. The FilmArray system (BioFire; Salt Lake City, UT) is a novel and completely integrated real-time multiplex PCR instrument (Figure 24.7a) that incorporates multiple single-plex PCR reactions in a disposable pouch (Figure 24.7b), [58,189]. Originally launched for the detection of respiratory viruses [132,186,189,196], with the capability to identify bacterial pathogens, the latest addition to their FDA-cleared menu includes a gastrointestinal (GI) panel for bacteria, viruses, and parasites, and the blood culture identification (BCID) panel for the detection of relevant bacteria and yeasts, as well as antimicrobial resistance bacterial genes. The BCID panel multiplexes 27 different genetic targets while the GI panel multiplexes 22. All NA’s are extracted and purified directly from the unprocessed sample. Since the FilmArray is both an integrated and a multiplex PCR/RT-PCR assay, the platform will be further described in the multiplex section below. Figure 24.7 (a) BioFire Diagnostics’ FilmArray instrument and (b) freeze-dried reagent pouches. (Courtesy of BioFire Diagnostics, Inc.) The FilmArray performs a nested multiplex PCR assay. During the nested PCR the FilmArray performs a single, large volume, multiplexed reaction with primers specific to several targets. Following the multiplex PCR reaction, an individual single-plex second-stage PCR reaction further amplifies the products that amplified during the first round of multiplex PCR. The FilmArray software analyzes and interprets the end-point melting curve of the amplicons. New panels in development include a meningitis/encephalitis panel and a lower respiratory tract pathogen panel. Although Luminex’s bead-based technology (Luminex Corp, Austin, TX) has been in use in clinical virology since the mid-2000s, release of the Luminex Gastrointestinal Pathogen panel (GPP) in 2012 combined viral, parasitic, and bacterial targets and was the first appearance of an FDA-cleared bead-based assay to include bacterial targets. Luminex xTAG technology assays are performed on the Luminex 100/200 instruments (Figure 24.8a) or the MAGPIX (Figure 24.8b), the latest version of the technology. The Luminex assays are composed of a multiplexed PCR reaction that amplifies the regions of interest in the target pathogen genes followed by treatment with exonucleases to remove excess nucleotides and primers. Subsequently, a primer extension step that is specific for the pathogen target that is being analyzed is included in the PCR reaction. The 5’ end of the primers is attached to a universal tag sequence. The 5’ universal tag sequence is hybridized to the complementary anti-tag sequence coupled to a particular xMAP bead set. After performing a wash step, detection is initiated. The hybridized beads are read by aspirating the assay samples, one after another, into the reader. The hybridized beads are carried by a microfluidic system in a stream of fluid through the measurement cuvette, where they are individually irradiated by dual reporter lasers. A red laser identifies each bead (or pathogen) by its color-coding, while a green laser detects the hybridization signal associated with each bead (indicating the presence or absence of a particular pathogen). Instrumentation reads the color-coded beads that attach to specific NA sequences, and results are analyzed by specific data analysis software [52,53]. Figure 24.8 (a) The Luminex 100/200 system and (b) the Luminex MAGPIX instrument are used for detection of targets downstream to multiplex PCR. (Images provided by Luminex Corporation.) Luminex xMAP technology is supported by the principles of flow cytometry and the major advantage of the technology is centered on the bead color-coding, which enables up to 100 possible separate interactions that can be analyzed simultaneously [52,105]. The entire procedure can be completed in approximately 5 h, making it a quick method to identify multiple genetic targets. Other significant advantages over traditional methods include: the versatility of one system to analyze several target types, the accuracy, the speed, and the standardization observed because of the reproducibility of high-volume production of xMAP microspheres within a single lot. The new Luminex instrument, the MAGPIX, is a compact instrument being launched to support bacterial panel testing. Luminex xTAG panels are available, as the xTAG Gastrointestinal Pathogen Panel (xTAG GPP), to detect Salmonella, Shigella, Campylobacter, enterotoxigenic Escherichia coli (ETEC), E. coli O157, Shiga-like toxin producing E. coli (STEC), and C. difficile toxin A/B, in addition to viruses and parasites associated with gastrointestinal disease. The current FDA-cleared GPP includes 11 targets in all. Additional targets,Vibrio cholerae and Yersinia enterocolitica are available but not in the United States at the time of this writing. The Respiratory Virus panel, which includes eight viruses and subtypes, is being updated to include bacterial targets. The multiplex aspect of the xMAP panels allows the identification of infections due to multiple pathogens, with rapid diagnostic results for diagnosis and patient management [13,150,164,180,255]. After Luminex and BioFire (formerly Idaho Technologies, now bioMérieux) entered the market with multiplex assays with more than ten targets, the second round of assays emerged, including GenMark Diagnostics (Carlsbad, CA) and Nanosphere (Northbrook, IL) instrument platforms. The eSensor technology (XT-8) (GenMark; Carlsbad, CA) promotes high assay specificity and sensitivity owing to competitive DNA hybridization and electrochemical detection of the post-PCR target sequence. The technology is not based on fluorescence or optical detection as are the first generation multiplex assays, thus the protocols do not require as many of the time-consuming washing and preparation steps required by earlier multiplexing technologies. The XT-8 systems (Figure 24.9a) can be used in tandem with up to three modules per computer for a throughput of 1–24 samples in random access mode. Figure 24.9 (a) The GenMark XT-8 system for detection of multiplex PCR products and (b) the test cartridge. (Images provided courtesy of GenMark Diagnostics.) The eSensor cartridge (Figure 24.9b) is preprogrammed with a specific test protocol, lot number, and expiration date on an internal memory chip. In the cartridge, the preamplified target DNA is mixed with the signal probe solution, and then analyzed via electrochemical detection. The first microbiological offering for eSensor technology was the Respiratory Viral Panel (RVP), an assay for respiratory virus detection and subtyping, which is a very sensitive RVP test. Based on independent studies these assays achieve up to 99.2% agreement with real-time PCR [187,188,204] and exhibit enhanced detection of coinfections, which can increase length of stay and may support changes in cohorting of the sickest patients [138]. The GenMark pipeline includes the HCV Genotyping Test (HCV 1a, 1b, 2a/c, 2b, 3, 4, 5, 6a/b) and the HCVg Direct test, with detection from serum or plasma [173,208]. New adaptation of the system toward one requiring less than the 60 min of hands-on time will make the GenMark system more easily adaptable to routine laboratory settings and is expected to be a fully integrated cartridge system with sample-to-answer workflows. Nanosphere’s Verigene (Northbrook, IL) system uses a gold nanoparticle probe technology, typically 13–20 nm in diameter that carries a defined number of target-specific oligonucleotides or antibodies utilized in two methodologies, array and amplification, depending on the specific assay. The nanoparticles support increased sensitivity compared to fluorophores. Light scattered from one nanoparticle is reported to be equivalent to the light emitted from one half million fluorophores. Automated NA purification and amplification is performed on the Verigene Processor SP (Figure 24.10a). Inside the Verigene Test Cartridges NA targets are hybridized to capture oligonucleotides on a microarray containing specific oligonucleotides and gold nanoparticle probes. Finally, signal amplification of probes occurs via a silver staining process with automated qualitative analysis performed on the Verigene Reader (Figure 24.10b). The first tier of assays available for the Verigene platform includes respiratory viruses, which are offered in amplification assays [3,107], and products for identification of select Gram-positive and Gram-negative pathogens from positive blood cultures, which are probe-based without target amplification [2,213]. Figure 24.10 Nanosphere’s Verigene SP system performs array-based detection of nonamplified or pre-amplified genetic targets by using (a) the Verigene SP processor and (b) the Verigene SP reader. (Courtesy of Nanosphere, Inc.) Another novel RUO application, the Unyvero system (Curetis AG, Germany) targets lower respiratory tract (LRT) bacterial pathogens using a highly multiplexed panel including multiple bacterial species and antibiotic resistance genes [106]. Transcription mediated amplification (TMA) is the synthesis of a DNA strand complimentary to a target NA, usually RNA [123]. The newly synthesized cDNA becomes the template for the subsequent in vitro transcription reactions. An excess of RNA is created and is used as the substrate for another round of transcription. Much like PCR, this process continues at an exponential rate and several million copies of the target can be created. The amplified target is then detected using various methods. Hologic/Gen-Probe (San Diego, CA) launched the PANTHER (Figure 24.11), the smaller version of the TIGRIS, a fully automated random-access TMA instrument for high-volume testing. Like the TIGRIS, which combines detection and decontamination into one instrument, the PANTHER also utilizes Gen-Probe’s target capture technology for sample processing, TMA amplification, and hybridization protection assay (HPA) [78]. The PANTHER provides the ability to test for four targets from one specimen using Gen-Probe’s APTIMA assays for Neisseria gonorrhoeae/Chlamydia trachomatis (GC/CT), human papilloma virus (HPV) [131], and Trichomonas vaginalis, providing a multi-targeted STD testing option. Currently the assays are limited to endpoint assays; however, the PANTHER has assays currently in development with real-time TMA capabilities to compete with quantitative PCR applications, specifically viral loads [245]. Figure 24.11 Hologic’s PANTHER instrument tests ThinPrep liquid cytology specimens via Aptima HPV mRNA-based assay is a nucleic acid amplified test that detects 14 high-risk strains of human papillomavirus (HPV) associated with cervical cancer and precancerous lesions. (Courtesy of Hologic, Inc.) Loop-mediated isothermal amplification of DNA (LAMP) is a novel method that is quickly impacting molecular detection of pathogens [170]. This method rapidly amplifies DNA under isothermal conditions with high specificity and efficiency. During the LAMP process, four to six different primers, specifically designed to recognize six or eight distinct regions on the target sequence, participate in amplification and detection of the target sequence, which can be completed in a single step [162,247]. The illumigene LAMP (Figure 24.12) amplification assays (Meridian Bioscience; Cincinnati, OH) use specific primers that continuously facilitate isothermal amplification producing the byproduct, magnesium pyrophosphate, in the reaction. The accumulation of magnesium pyrophosphate results in a precipitated solution. The resulting turbidity from the precipitate can be detected by the instrument, which functions as both an incubator and reader [156]. When the pathogen, specifically the gene target of interest, is not present, precipitate does not form and the amplification reaction is negative. When the gene target is present, the resulting amplification produces a turbid solution and a positive result. The illumigene C. difficile assay detects the pathogenicity locus (PaLoc) of toxigenic C. difficile by targeting a region of tcdA, with results available in about 1 h [113,114,124,168,169]. The illumigene test menu has expanded to include Group A streptococci, B. pertussis and Mycoplasma pneumoniae [5,124,197]. Figure 24.12 Loop-mediated isothermal amplification of DNA (LAMP assay) can be performed on Meridian’s Illumigene instrument. (Courtesy of Meridian Bioscience, Inc.) The New Alere i instrument (Alere; Waltham, MA) uses a semimanual process and visual touchscreen-driven process for isothermal amplification of influenza A and B genetic targets. The Alere i produces results in 15 min from samples such as nasal swabs and swabs in viral transport media. The instrument is designed with a small footprint for use in small throughput point-of-care settings. Isothermal amplification can be performed without the use of thermal cyclers in a wide variety of laboratory conditions; therefore, the isothermal nucleic acid amplification technology (INAAT) market is growing. Please consult current literature and company web sites for further information. Peptide NA (PNA) probes are fluorescent probes attached to PNA molecules that have a noncharged polyamide (peptide) backbone as opposed to the negatively charged sugar-phosphate backbone of DNA and RNA. PNA probes have the same nucleotides (adenine-A, guanine-G, cytosine-C, and thymine-T) and follow the same base-pairing rules allowing them to bind normally to complementary sequences of NA. There are several advantages to the structure of PNA probes over NA probes, namely: (i) PNA probes bind more strongly during the hybridization reaction than a DNA probe – PNA chemistry hybridizes more readily to the target of interest due to very small dye-labeled probes, approximately 12–20 nucleotides in size; (ii) The neutral charge of the PNA backbone reduces natural repulsion with negatively-charged backbones (i.e., DNA), therefore they bind tightly; (iii) greater specificity as a result of more precise binding to the target and exquisite base-discrimination; and (iv) PNA probes provide greater sensitivity – they add very low background with better visualization of the fluorescent signal. AdvanDx, Inc. (Woburn, MA) commercially markets FDA-cleared PNA FISH (fluorescent in situ hybridization) kits for clinical diagnostic use and identification of S. aureus, Enterococcus spp., Mycobacterium spp., E. coli, Klebsiella pneumoniae, and Pseudomonas aeruginosa, among other targets, including Candida spp. [32,69–71,93,154,155,239]. More cost-effective than PCR techniques, PNA FISH does not require extensive knowledge or experience in molecular techniques, since the workflow is much like routine immunofluorescence staining procedures. The chemistry of PNA FISH probes make this technology just as sensitive but more specific than Gram staining and direct fluorescence staining [239]. Because the PNA FISH kits are relatively easy to use, they are now widely accepted as a method for rapid identification of bloodstream pathogens. The rapid identification of bloodstream pathogens via PNA FISH may be the most widely studied of all rapid methods in terms of outcomes [27,69–71]. Originally the PNA FISH turnaround time was about 1.5 h; however, the next generation of PNA FISH, called QuickFISH (Figure 24.13), eliminates the wash step, thus reducing the assay time from 90 to only 20 min after the blood culture flags positive [30,44,45,145]. Limitations of PNA FISH are the inability to test multiple pathogens and the absence of complete antibiotic susceptibility information [68]. Figure 24.13 AdvanDx QuickFISH System offers results in 20 min of positive blood culture with 5 min of hands-on time. (Courtesy of AdvanDX) Matrix assisted laser desorption ionization time-of-flight (MALDI-ToF) methods leverage the high sensitivity of mass spectrometry (MS) with linkage to large microbial databases, to provide users with species-specific spectra that can be used to reproducibly identify microorganisms. Analysis of whole cells was first proposed in 1975 to study the biomarker profile of various bacterial species following pyrolysis-MS for low molecular weight products [6]. However, it was not until 1996 that the first MALDI-ToF/MS experiment was successful for identifying bacteria directly from whole colonies based on protein biomarkers [40,97]. A great number of developments have been made over the last decade on whole-organism MALDI-ToF/MS. The protein biomarkers that are measured in MS of microorganisms are highly expressed proteins responsible for housekeeping functions, such as ribosomal components, chaperones, and transcription/translation factors [38]. Relying on identification of protein profiles (generally measuring mass to charge ratios (m/z) between 2 and 20 kDa), MALDI-ToF/MS derives its information from profiles of highly conserved bacterial proteins. For MALDI-ToF, the protein profiles are generated from direct ionization of an intact colony or a bacterial protein extract, after manual extraction. Identification occurs after correlating an isolate’s spectral signature to a database of spectra collected from reference strains (Figure 24.14). Figure 24.14 Schematic for MS analysis of ionized microbiological isolates and clinical material. Once appropriately processed, samples are added to the MALDI plate, overlaid with matrix and dried, then the sample is bombarded by the laser. This bombardment results in the sublimation and ionization of both the sample and matrix. These generated ions are separated based on their mass-to-charge ratio via a ToF tube, and a spectral representation of these ions is generated and analyzed by the MS software, generating an MS profile. (Copyright © American Society for Microbiology [Clinical Microbiology Reviews, Volume 26, Number 3, 2013, pp. 547–603] doi: 10.1128/CMR.00072-12.) In its current form, MALDI-ToF/MS requires cultivated microbes and a small quantity of matrix (typically less than 1 μg/sample). The matrix and target spotting plates/slides are the primary consumables and are relatively inexpensive in disposable or reusable formats. Ongoing costs include the equipment maintenance agreements. MALDI-ToF/MS is widely used, for its high accuracy, low consumable cost, and speed of analysis. A typical process consists of growth of bacteria or yeast, colony selection and placement on a target, addition of matrix, and analysis with MALDI-ToF/MS. Mass spectrometry identification has diverse possibilities, as it is a fast and sensitive method to measure proteins, lipids, and other targets that does not require prior knowledge about the organism. Several reviews of MALDI-ToF/MS technology are available [15,38,49,101,128,190,214]. Commercial MS systems exist that can integrate with traditional antimicrobial susceptibility systems. Among them, the MALDI Biotyper from Bruker Daltonics (Figure 24.15a) is among the most widely tested and has proven accuracy in the identification of bacteria [135]. The system uses a 48-spot MALDI target plate (Figure 24.15b) and is partnered with the Becton Dickinson Phoenix System or the Siemens MicroScan, which supply antimicrobial susceptibility results that pair with bacterial identification. Evaluations of the system show high accuracy for rare or fastidious bacteria, yeasts, and fungi, which represent a challenge to clinical laboratories using phenotypic identification evaluation due to their limited biochemical reactivity [1,141,142,207]. Figure 24.15 (a) The Bruker MALDI Biotyper CA System includes a bench-top microflex MALDI-ToF mass spectrometer, software, reagents, a library of microorganism reference spectra, and (b) a 48-spot MALDI target plate. (Courtesy of Bruker Daltonics.) A few reports show promise for the identification of microorganisms using MALDI-ToF/MS without subculture [66,160,194,217,240], but the methods were limited by the need for a large number of cells. These adaptations showed successful identification for only approximately 80% of blood cultures. A promising new technology, the MALDI Sepsityper system (Bruker Daltonics), aims to identify bacteria and yeast directly from positive blood-culture bottles [118,145,217,271]. Another MALDI-ToF system, the bioMériuex Vitek MS (Figure 24.16a), exhibits similar capabilities; no statistically significant difference was observed between the two platforms for organisms clinically encountered [35]. A multicenter clinical trial group published several reports to document the high degree of accuracy supported by Vitek MS version 2.0 [23,73,147,256]. The accuracy of Vitek MS was high when compared with the reference method (DNA sequencing) and provided an accurate, species-level identification for 96% of 226 isolates; an additional 1% were accurately identified to the genus level [23,137,205]. Similarly, of 651 isolates analyzed, 91.2% exhibited the correct anaerobic species identification based on 16S rRNA gene sequencing: at the genus level, the agreement rate increased to 92.5% [73]. For yeast, compared to sequence analysis of the D2 region of the 26S rRNA gene, 823 isolates (96.6%) were correctly identified to the genus level and 819 isolates (96.1%) were correctly identified to the species level [256]. The Vitek MS uses middleware name “Myla” to connect to the Vitek 2 (Figure 24.16b) and uses a 48-well disposable array (Figure 24.16c). Figure 24.16 (a) The bioMériuex Vitek MS MALDI-ToF technology, depicted here with (b) the Vitek 2 system for susceptibility testing, couples with reagents, a comprehensive database of clinically relevant species, and (c) a disposable array. (Courtesy of bioMérieux.) While fundamentally equivalent in performance, the Vitek MS differs from the Bruker Biotyper in its instrumentation scoring algorithm and its databases. Both instruments are supported with an FDA-approved database and both are partnered with automated systems for antimicrobial susceptibility testing, but both have limitations in that the databases need to be expanded to include rare human pathogens. Neither system currently performs antimicrobial susceptibility testing (AST); neither have high accuracy from direct specimens, with the exception of the Bruker Sepsityper. With SepsiTyper, positive blood cultures are purified and subjected to rapid identification using MALDI-ToF/MS [134]. The need for growth of organisms with isolated colonies is still required in order to obtain an accurate result because the technique’s ability to resolve polymicrobial mixtures is lacking. Additionally, a high number of bacterial cells are required for identification, such that a whole intact colony is typically used for analysis, limiting the ability to rapidly identify microorganisms directly from biological fluids where the bacterial count is expected to be relatively low. Research is currently being performed to mitigate some of these requirements. Until accurate determinations of resistance factors can be made, parallel culture-based recovery of positive cultures will most certainly be required for the foreseeable future for antimicrobial susceptibility testing [217]. The major barrier to implementing MS-based technologies to the clinical laboratory is the cost associated with the capital purchase of a dedicated mass spectrometer [259]. Thus, as is true for most newly emerging technologies, these methods may be limited to larger reference laboratories until the cost benefit is fully evaluated or until smaller more affordable bench-top options are developed. Nevertheless, the potential for high analytical sensitivity, accuracy, and broad-based applicability of MS-based platforms have the potential to change the practice of clinical microbiology in the future and offers the promise of off-setting some portion of the complexity and workload in clinical microbiology. Sequence-based identification, strain typing, and comprehensive isolate fingerprinting can be utilized for the tracking and control of pathogenic organisms; therefore molecular methods like sequencing have been a widely used tool for epidemiological fingerprinting of isolates important to public health. In 1995, the first two complete DNA sequences of the bacterial genomes Haemophilus influenzae and Mycoplasma genitalium were revealed by scientists at The Institute for Genomic Research (TIGR), followed in 1996 by the first complete genome sequencing of an Archaea, Methanococcus jannaschii. Since then automated sequencing using fluorescent chain-terminating nucleotides during elongation of a DNA template (referred to as Sanger sequencing) has made it possible to rapidly sequence the genomes of many pathogens and environmental microorganisms both in research and diagnostic settings. A major advantage of sequencing is it allows for the comparison of genomes identifying differences between virulent and avirulent medically important pathogens. The comparison data generated at the genomic level of a pathogenic species can provide information about host or tissue specificity and antimicrobial resistance patterns. The clinical applications of sequencing extend far and wide, including but not limited to, identification of cultured and nonculturable isolates, pathogenicity and drug resistance, strain typing in outbreak situations, and emergence of new drug-resistant strains. Pyrosequencing is a DNA sequencing method that detects released pyrophosphate as DNA polymerase acts on a target sequence. Pyrosequencing assays have been developed for several applications including genotyping, single nucleotide polymorphism (SNP) detection, and microorganism identification. Pyrosequencing is real-time sequencing and thus can be used for not only microbial species identification but for the discrimination of point mutations that confer antimicrobial resistance. There are several major advantages to pyrosequencing (PPi): it is high throughput, sensitive, rapid, simple, customizable, and highly specific [109,110,195,274]. The principle of the pyrosequencing reaction relies upon annealing of a target primer to a single stranded template. After binding, DNA polymerase acts to elongate the sequence, much like PCR. As the polymerase adds deoxynucleotides, in a predefined pattern, the primers extend and release inorganic pyrophosphate as part of the synthesis. Inorganic pyrophosphate is converted into ATP by ATP-sulfurylase. The accumulating ATP is utilized by firefly luciferase to produce light. The pattern of light signals, correlating to the added nucleotide, is a true representation of the sequence that is being analyzed. The amount of light generated by the reaction is directly proportional to the PPi produced or the number of nucleotides that were incorporated into the newly generated sequence. Any nucleotides that are not incorporated into the new sequence are enzymatically degraded. The sequence generated by pyrosequencing is quantitative in nature. Select pyrosequencing methods utilize a solid-phase template preparation of streptavidin beads that interact with biotinylated 5’ ends of single-stranded DNA as opposed to the enzyme-based template preparation. The reaction is rapid, less than 2 h to result, and DNA extracted from multiple microbial species in a single specimen can be sequenced in the same assay batch. Still, disadvantages to the technology consist of the method being relatively hands-on, labor intensive, and open to potential contamination events. In addition, the sequencing reaction is only able to produce sequence for short targets, in some cases < 100 nucleotides. Qiagen PyroMark (Qiagen Inc.; Valencia, CA), a commercial system (Figure 24.17), is available in three system formats, Q24, Q96, and Q96MD, while Roche markets the GS Junior and GS Flex pyrosequencers. Figure 24.17 QIAGEN’s PyroMark Q24 pyrosequencer. (© Qiagen, all rights reserved.) A novel combination of automated microscopy paired with electrophoresis of microbes, and FISH genetic probes, the Accelerate instrument (Figure 24.18a; Accelerate Diagnostics, Tucson, AZ) utilizes both genomic and phenotypic detection technologies to significantly decrease time to result while sustaining high sensitivity and specificity. This process will occur on a circular cartridge (Figure 24.18b). Although not yet FDA cleared as of this writing, a recent report details use of the ID/AST prototype to correctly classify Klebsiella pneumoniae
Clinical Microbiology: Looking Ahead
24.1 Introduction
24.2 Connectivity between extraction and amplification platforms
24.2.1 Requirements for upstream nucleic acid extraction
Kit/platform
Manufacturer
Technology
Number of samples/total time
Specimen type(s)
m2000sp*
Abbott
Magnetic silica particles
24–96/2–2.5 h
Serum, plasma, vaginal, rectal, nasopharyngeal swabs, stool
NucliSENS
EasyMAG
bioMérieux
Proprietary “Boom” technology with magnetic silica
24/batch
Most sample types (plasma, serum, whole blood, stool, respiratory samples, etc.)
50–1000 μL
BioRobot EZ1
Qiagen
Magnetic silica particles
6/20 min
Dermal, genital, nasal swabs
QIAcube
24/1.5 h
QIAagility
96/1.5 h
QIAsymphony*
96/2 h
MagNA Pure LC
Roche Applied Science
Magnetic silica particles
32/90 min
Serum, whole blood, plasma, stool, sterile body fluids, respiratory tract specimens, dermal, genital swabs
MagNA Pure Compact
8/30 min
COBAS Ampliprep*
96/2 h
Maxwell
Promega
Paramagnetic particles
16/45 min
Blood stain cards, buccal swabs, whole blood, tissue
24.3 Polymerase chain reaction and RT-PCR: detection and characterization
24.3.1 Real-time PCR
24.3.2 Recent innovations for reverse-transcription and real-time PCR assays
24.3.2.1 Simplexa assays
24.3.2.2 IntelligentMDx assays
24.3.3 Digital PCR
24.3.4 Integrated PCR assays
24.3.4.1 GeneXpert assays
24.3.4.2 Focus Simplexa assays
24.3.4.3 The FilmArray
24.3.5 Multiplex PCR/RT-PCR
24.3.5.1 FilmArray
24.3.5.2 Bead-based assays
24.3.6 Second generation multiplexes
24.3.6.1 GenMark eSensor
24.3.6.2 Nanosphere Verigene
24.3.6.3 Curetis Unyvero
24.4 Other amplification methods
24.4.1 Transcription mediated amplification
24.4.2 Loop-mediated isothermal amplification of DNA
24.5 Probe technology
24.5.1 Peptide nucleic acid–fluorescent in situ hybridization
24.6 Mass spectrometry
24.6.1 Matrix assisted laser desorption ionization time-of-flight spectrometry
24.6.1.1 Bruker MALDI Biotyper
24.6.1.2 bioMériuex Vitek MS
24.6.2 Limitations of MS-based technologies
24.7 DNA sequencing
24.7.1 Capillary sequencing
24.7.2 Short read pyrosequencing
24.8 Emerging technology
24.8.1 Automated microscopy: ID/AST
Stay updated, free articles. Join our Telegram channel
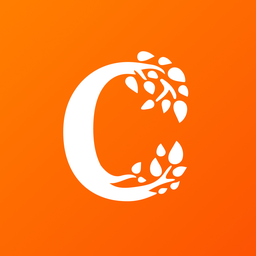
Full access? Get Clinical Tree
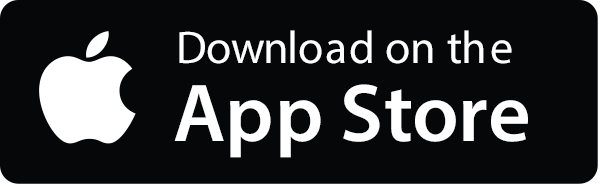
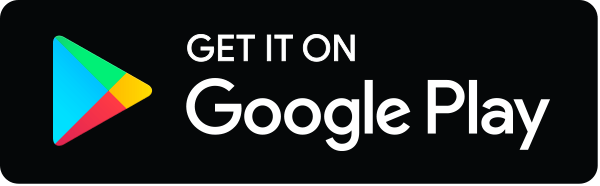