Dynamic Materials Mimic Developmental and Disease Changes in Tissues
Material Science Program, University of California–San Diego, La Jolla, CA, USA
Material Science Program, Department of Bioengineering, Sanford Consortium for Regenerative Medicine, University of California–San Diego, La Jolla, CA, USA
2.1 Introduction
A growing body of evidence suggests that cells are influenced not just by soluble growth factors, but also by the extracellular matrix (ECM), a three-dimensional (3-D) fibrillar material to which cells adhere [1,2], and its intrinsic properties [3], for example, structural [4,5], biochemical [6], and mechanical [1,7]. Not only are cells influenced by intrinsic aspects of the ECM, they can also modify ECM to change its properties [8, p. 226] and thereby direct the behavior of adjacent cells [3]. For example, the ECM is composed predominantly of three major proteins, that is, collagen, fibronectin, and laminin, and each has a very specific distribution in the body [8, pp. 349–359] that contributes to developmental process, for example, fibronectin is made by mesodermal cells for the endoderm to use as a substrate on which to migrate during gastrulation [9]. Cells also establish an ECM to influence morphology [10], stiffness [11], lineage [1], and durotaxis (directed motility as a result of a change in stiffness in the microenvironment) [12,13] of adjacent cells. By better understanding the dynamics of these changes, we will be able to engineer materials that will better mimic the dynamic nature of cells and tissues in vivo. While many aspects of the matrix change with time as cells remodel it, this chapter will mainly focus on the cell and dynamic tissue stiffness and questions of when, why, and how they change. We will do this in the context of several examples where we will detail how the environment changes with time and how that impacts cell behavior.
Prior to that, it is important to first understand the molecular mechanisms of how and why cells respond to physical stimuli, including passive stimuli such as stiffness. Cells perceive and feel the passive properties of their surroundings, for example, matrix stiffness, through physical signals it receives from transmembrane receptors called integrins, which are converted into biochemical signals that the nucleus or other organelles can interpret in a process called mechanotransduction. Mechanisms proposed to act within or surrounding perinuclear regions of the cell can cause nuclei to get stiffer as stem cells differentiate [14] and such changes can be dependent on cytoskeletal coupling to the nucleus via SUN- and LINK-domain-containing proteins [15]. Many other mechanisms have been proposed within focal adhesions, which are dynamic cell–matrix linking structures composed of clustered integrins located at the end of actin stress fibers [16,17]. They are integral in coordinating cell migration among many other processes [18]. Focal adhesion assembly and disassembly allows for cell movement along the matrix and is regulated by the tyrosine kinase called focal adhesion kinase (FAK) which is located at cell adhesion sites [16,18,19]. The expression of FAK has shown to be upregulated in metastatic tumors [16,20,21] and is essential in fibroblast spreading [22] and migration [23]. Rho GTPase-dependent cell signaling changes [13,24] directly impact actomyosin function and thus regulate contractile force [25,26], which plays a prominent role in cell spreading and adhesion [27]. Changes in stretch-activated channels to regulate calcium influx have been proposed and could also directly alter actomyosin function [15]. However, what directly transmits actomyosin contractions and relays signals back to the cell are cell–matrix adhesions, which have been an area of great interest for mechanotransduction [28]. Within these complexes, integrins bind to matrix outside the cell membrane, and on their cytoplasmic side, bind to actin via talin, which itself has many regions that unfold with force to induce signaling events [29]. Talin unfolds on application of 12 pN force, unveiling vinculin binding sites, which have mechanical/chemical signaling implications [15,29,30]. Proteins within this complex that change confirmation under force and expose cryptic sites have been termed molecular strain gauges [15]. Transduction within these complexes can be bidirectional where the cell generates a chemical signal in reaction to the mechanics of the ECM, which ultimately reinforces the attachment strength of the adhesions on ECM proteins [31]. This process is highly dynamic as the forces subjected to an adhesion fluctuate with both space and time, even within a single adhesion [32], and thus the intensity of signaling itself can scale with the stability of focal adhesions [33]. Through these different mechanisms, cells can feel the mechanics of their microenvironment and can respond by changes that are evident in cell morphology, motility, and lineage.
With these sensing mechanisms in mind, we now review the scaffold types and materials employed for cell culture and tissue engineering, both synthetic and natural. Unlike other reviews herein, we will pay particular attention to the dynamic nature of the scaffolds and the influence that that has on cell form and function.
2.2 Cell Scaffolds, Their Intrinsic Properties, and Their Effects on Cells
A biomimetic environment that promotes cell adhesion, proliferation, and directed lineage is very important to developing the appropriate cell behavior in vitro. However, cells are often cultured in vitro on substrates that are less biomimetic, for example, tissue culture plastic. Rigid materials do not simulate the in vivo microenvironment of a cell [34,35], and hydrogels are commonly substituted because of their capacity to mimic many intrinsic properties of the ECM by their high water content, mechanical properties, structure, adhesivity (when functionalized), and so on [36]. For example, mechanical properties, specifically Young’s modulus (EY; measured in pascals, Pa) or stiffness (as it is referred to in biological contexts), of ECM is known to have a strong influence on the lineage of stem cells; polyacrylamide (PA) hydrogels with a stiffness that mimics muscle encourages mesenchymal stem cells (MSCs) to begin to commit toward a myogenic lineage in the presence of serum-containing media [1]. Hydrogels are made from a number of different sources, both naturally derived, for example, glycosaminoglycans (GAGs), and synthetically produced polymers. In this section, we will review the basic types of polymer, the unique biological, chemical, and mechanical properties each offers, and the resulting effects on cells.
2.2.1 Natural Polymers and Their Properties
A number of polymers that are used for cell scaffolds are naturally derived from biological sources and can be found in the body. These biopolymers are naturally biocompatible, can form many different structures depending on their assembly, and have many different functions that augment their role as a cell scaffold. Collagen is a biopolymer that comes from over 100 different genes, but assembles into 29 distinct types, each with its own distribution and signaling properties in vivo [8, pp. 5–22,37]. Gelatin, an irreversibly hydrolyzed, denatured form of collagen [38], is often derived from mammalian skin and used in hydrogels when cross-linked by chain entanglement at higher temperatures. Other matrix proteins, for example, fibrin [39], require cells to aide in their assembly, but together, these polymers are very flexible with low persistence lengths and small globular structures Unmodified natural polymers are typically mechanically inferior to synthetic polymers, in terms of stiffness, achieving an EY of up to only a few kilopascals (kPa) [40], which limits the types of tissue it can emulate. Conversely, polysaccharides, including anionic alginate [41], GAGs, and hyaluronan (HA) [42–44], have large amino sugars, making them less flexible. Via chemical functionalization, these polymers can be covalently linked and can provide properties for cells that more appropriately mimic native niches. For example, HA polysaccharide structure has several functional groups that can be chemically modified, through mechanisms such as thiolation [42] and methacrylation [43,44], allowing one to tune the mechanical properties toward a particular application. HA also acts as a ligand for CD44, allowing for cells to adhere without the use of adhesive peptides [45,46]. Given the diversity of intrinsic properties from their many ligands to varied stiffnesses, natural polymers can provide many different microenvironments, often in 3-D, but their ability to predictably and reproducibly create these niches is not the same as synthetic polymers.
2.2.2 Synthetic Polymers and Their Properties
Synthetic polymers are advantageous for cell scaffolding applications because of the various ways in which they can be polymerized, as well as the larger range of intrinsic properties that can be achieved, for example, different structures (fibers [47], foams [48], hydrogels [1], etc.) and stiffnesses among others [40]. Synthetic polymers are typically biologically inert and require the use of adhesive peptides to allow for cell attachment [40]. For example, polyethylene glycol (PEG) [49,50], polydimethylsiloxane (PDMS) [51,52], and PA [35,53] hydrogels do not have cell-binding receptors, and therefore require a protein layer to encourage cell adhesion. Additionally, PEG [54], PA [35,55], and PDMS [56] are capable of reaching a variety of biological stiffnesses (Figure 2.1). However, most synthetic polymers are composed of very small monomers, generating a polymer with smaller pore size, which prevents any cell migration through the polymer, which limits their usage in cell encapsulation studies [40].

PA and PEG, when chemically modified with acrylate groups to form polyethylene glycol diacrylate (PEGDA), for example, can be polymerized by different free radical addition reaction: photopolymerization via an ultraviolet (UV) photoinitiator such as Irgacure 2959 (BASF—The Chemical Company, Minden, Germany) [43,44,57,58] or chemical methods using ammonium persulfate (APS) in combination with N,N,N′,N′-tetramethyl-ethylenediamine (TEMED) [12]. PDMS polymerizes by a curing process at 60°C in the presence of a cross-linker, and can reach stiffnesses in the megapascal (MPa) range [56].
2.2.3 The Effects of Scaffolds on Cells
It is important to recognize that the intrinsic properties mentioned in previous parts of this section are presented initially to cells. As the cells interact with the material, as described below, the cells are not only receiving signals from the scaffold, but they also remodel the scaffold. This dynamic state is often referred to as dynamic reciprocity [59] and can be summarized by the idea that while intrinsic ECM properties influence cells, the cell in turn remodels the matrix, thus shaping the signals that it receives. For progenitor cells, this process regulates many behaviors as well as cell fate. While this local remodeling presents active changes to the cell that is assembling or changing the matrix, the other cells around it respond to the passive changes that it induces. These sections to follow highlight how cells respond to passive matrix properties, but ultimately, we are concerned with how their collective actions together result in developmental changes that can be mimicked by dynamic matrices.
2.2.3.1 Stem Cells
As stated previously, one of the ECM’s intrinsic properties, stiffness, plays a significant role in determining cell lineage [1,60,61]. It has been shown that stem cells are highly perceptive to the mechanics of their microenvironment [1,7]. MSCs cultured on PA hydrogels of different stiffnesses expressed different lineage-specific markers. β3 Tubulin, a neurogenic marker, MyoD, a myogenic marker, and CBFα1, an osteogenic marker, were expressed on 1 kPa (soft), 11 kPa (intermediate), and 34 kPa (stiff) hydrogels, respectively (Figure 2.2) [1]. Stiffness has shown to have an important influence on neural progenitor cell fate as well. Neural stem cells (NSCs) cultured on hydrogels with stiffness between 0.1 and 10 kPa exhibited different neural lineages; neuron differentiation decreases with increasing hydrogel stiffness, while glial differentiation increases [62]. Likewise, myogenic differentiation is affected by scaffold stiffness. MSCs undergo myogenic differentiation in the presence of transforming growth factor beta (TGF-β), a promoter of myogenic differentiation, and also on hydrogels with stiffness more similar to firm muscle, for example, 11 kPa. Cells also exhibit more cell spreading, proliferation, and stress fibers [63].

Cells also respond to different spatially defined patterns on the ECM [64–66]. Using microcontact printing to pattern the scaffold with ECM proteins permits cells to adhere to specific patterned regions of the ECM [66,67]. This allows for control of cell morphology, which in turn controls cell traction forces, and ultimately determines cell lineage. Using different shapes and aspect ratios of micropatterns allows one to finely tune stem cell lineage, by mimicking the shape of the desired cell type. For example, using a star shape without additive media pushes cells toward an adipogenic fate [64]. This idea has shown to be a viable method to creating cell constructs, such as myotubes [68–71]. Hydrogels with a myogenic elasticity, coated with collagen strips, have shown to develop fully formed myotubes [70], while others have used mechanically patterned acrylamide hydrogels with distinct soft and stiff regions that promote durotaxis, cell alignment, and fusion, resulting in myotube formation of adipose-derived stem cells (ASCs) (Figure 2.3) [68]. It is hypothesized that these cellular responses are likely due to the remodeling of the cell cytoskeleton and focal adhesions in reaction to the mechanics and features of the microenvironment [61].

Nanopatterned topographical features on hydrogels, as well as nanopores, have been shown to influence cell lineage [72–74]. Electrospinning polymer cell scaffolds have shown to be an effective option for creating a cell-responsive nanoporous surface. MSCs cultured on chitosan electrospun scaffold align along the fibers and adopt a myogenic lineage [73], while MSCs cultured on electrospun methacrylated hyaluronic acid express increased chondrogenic markers with increasing scaffold adhesivity [74]. However, electrospun samples must have appropriate pore size; when too small, cells cannot infiltrate the nanosized pores [75,76]. Other methods such as using nanopatterned scaffold have emerged as an effective way of differentiating stem cells. MSCs plated on PDMS scaffolds, patterned with 350 nm wide grids, have shown to align along the grids and undergo neurogenesis [72], while PDMS patterned with a 600-nm wide grating similarly observed embryonic stem cell (ESC) alignment [77], indicating the importance of mechanics and topographical features on cellular activity.
2.2.3.2 Cancer Cells
Likewise, the ECM can significantly influence the onset of cancer and tumor metastasis. Malignant transformation begins in normal tissue at a healthy stiffness, but as part of the transformation to a tumor, overproduction of ECM, that is, fibrosis, often results. Thus the onset of cancer usually occurs with tissue stiffening, leading to enhanced integrin signaling and aberrant cell growth [78–80]. Though this is a hallmark of breast cancer in that you can manually palpate mammary tissue and feel a stiff lump, fibrosis occurs in many situations including in cirrhosis of the liver and fibrosarcoma. ECM stiffening directly promotes metastasis of the tumor and indirectly assists in tumor angiogenesis and inflammation [79]. A stiffer ECM can also disrupt the vasculature from normal tissue and leads to tumor growth [81].
While the influence of stiffness on tumor progression is still a relatively new topic and is likely very dynamic, the effect of step changes in static ECM stiffness on mammary epithelial cells has been extensively studied. In healthy mammary tissue, mammary epithelial cells develop into 3-D hollow spheres called acini. There are two stages to the tumor development; premalignancy and malignancy. When the tumor enters the premalignant stage, the ECM stiffens threefold from healthy tissue, from approximately 100 to 350 Pa [82]. Once the tumor becomes malignant, the ECM undergoes a 10-fold stiffness increase to 1500 kPa [35,82]. During this stage, the acinar structure of the mammary epithelial cells fail to develop and instead, the polarity is compromised. The cells can become highly invasive cells that mirror breast cancer cell metastasis (Figure 2.4) [35]. The composition of the ECM can also play a role in acini formation in causing a redistribution of the actin filaments, effecting morphology and acini formation [83].

2.2.3.3 Embryonic and Progenitor Cells
The surrounding microenvironment plays a critical role in regulating ESC fate during embryogenesis [84]; cells are destined to either undergo self-renewal, that is, the process where two identical daughter cells are generated from a single parental cell, or develop into mature tissues via differentiation. For the latter case, tissues result from tightly controlled spatial and temporal presentation of growth factors to comprise the cell niche, and from this extracellular set of signals, stem cells must integrate their response [85,86]. Examples of such developmental programs can be found for virtually every tissue, and such regulation has even been shown to reprogram the fate of cells to transform from one lineage to another [87]. Similar phenomena are seen in different species including, mice, chicks, and zebrafish, where notch signaling is vital in deciding cell fate [88]. Notch signaling describes the time-sensitive developmental pathway that is responsible for guiding stem cell fate at different stages in development [89,90]. Notch has been demonstrated in the Drosophila and vertebrate models [91,92] and has shown to promote neural differentiation in ESCs [92]. It has also been implicated in central nervous system development by guiding neural progenitor fate to either glial or neural cell development [93].
2.3 ECM is a Dynamic Tissue
ECM is dynamic by nature; matrix is constantly being remodeled and maintains a balance of structural synthesis and destruction [8, pp. 179–251]. ECM is degraded by specific matrix metalloproteinases (MMPs), which cleave structural proteins such as collagen, resulting in degradation, and if not immediately filled by the cell, new matrix is likely synthesized to replace the degraded material. This process starts in the cytoplasm where new ECM components are synthesized and then secreted from the cell to rebuild the matrix [94]. When this balance of construction and destruction is disrupted, cellular diseases result when ECM is overproduced, resulting in a stiffer matrix and fibrosis. Excessive or aberrant production of MMPs is known to be a key contributor to tumor progression as well as heart disease [95,96]. Specific MMPs are known to supply critical growth factors to promote tumor growth and to degrade the ECM proteins, which results in tumor metastasis [97]. Likewise, there is an increased level of MMPs (collagenase and gelatinase) during the early stages of heart failure and in atherosclerotic plaque formation [98,99].
While dynamic stiffening characterizes many critical disease processes, for example, cancer metastasis [35,78,80,82] and myocardial infarction/heart failure [100], it is also critical in the developmental stages of embryogenesis [101–103]. In this case, however, ECM degradation is an integral part of the remodeling process, as it allows for cells to incorporate and regenerate the necessary ECM proteins to construct their own ECM [82]. In the heart for example, this requires the downregulation of laminins and fibronectin in favor of type 1 collagen, which has been shown to assist in stiffening chick myocardium as it develops from E3 to E14 in vivo
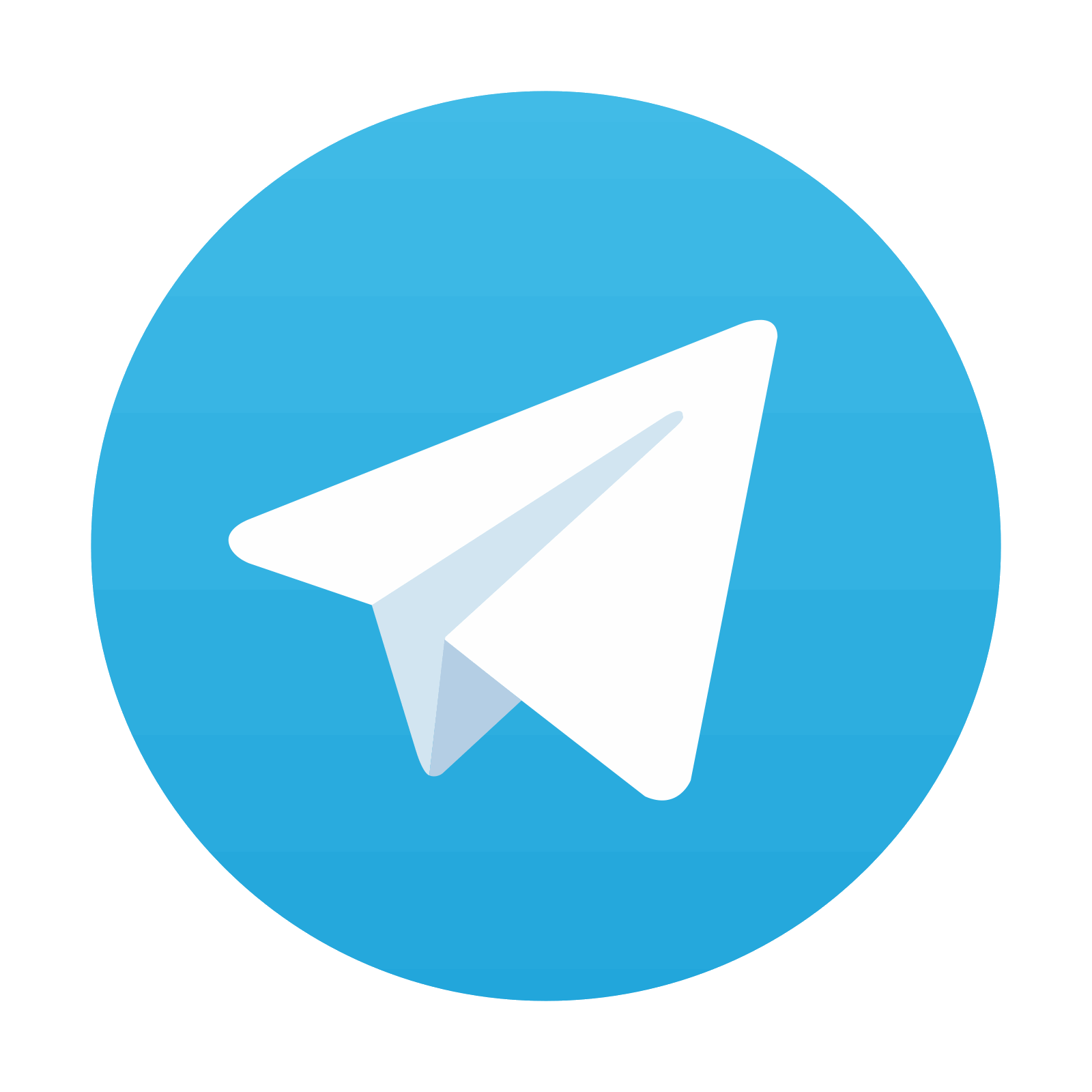
Stay updated, free articles. Join our Telegram channel
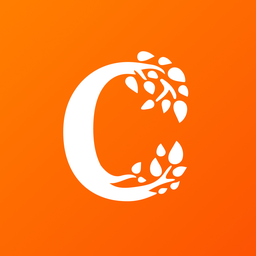
Full access? Get Clinical Tree
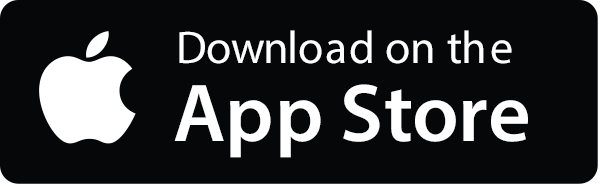
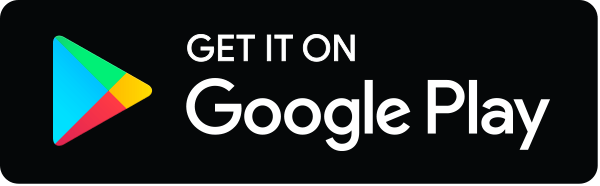