Immunomimetic Materials
J. Crayton Pruitt Family Department of Biomedical Engineering, University of Florida, Gainesville, FL, USA
18.1 Introduction
The development of biomimetic/bio-inspired materials is rooted in the fields of tissue engineering and regenerative medicine. Synthetic scaffolds are often used to provide mechanical support and time-limited architecture for neotissue regeneration in vivo. Although synthetic scaffolds demonstrate favorable chemical and physical qualities, their lack of bioactivity is a limitation. Conversely, materials from natural sources with innate biomolecular recognition ability typically lack customizability, in addition to concerns regarding sterilization and immunogenicity. Biomimetic materials are a relatively new class where synthetic materials are adapted to possess biological motifs that direct specific responses at the molecular and cellular levels. These hybrid materials combine advantageous traits of both levels to provide well-controlled physical and mechanical properties, as well as biochemical and biological cues that influence material–cell communication.
Degradable biomimetic materials, as an example seen in numerous applications, aim to mirror many characteristics of the extracellular matrix (ECM) found during normal tissue formation and wound healing [1, 2]. The ECM plays a critical role in the morphogenesis, homeostasis, and regeneration of tissues throughout the human body. It is an ordered three-dimensional array of structural and functional biomolecules that differs in microstructure and biological activity for each tissue and organ. The ECM is secreted by the local population of cells and not only bestows structural integrity but also drives cell and tissue phenotype by sequestering and releasing biochemical signals in a spatially and temporally dependent manner. Furthermore, the ECM is a dynamic structure constantly undergoing remodeling due to environmental forces such as mechanical loads, oxygen debt, and pH [3]. One of the major biomimetic material strategies involves incorporation of ECM characteristics into synthetic structures to develop biointeractive constructs.
In addition to mimicking characteristics of the ECM in which cells reside, recapitulation of certain features associated with cells themselves has also served as inspiration for biomaterial scientists. One of the most basic aspects of cells is that of compartmentalization. Selective isolation of biomolecules allows for exquisite control of metabolic reactions in a spatiotemporal context required for cell functionality. While accomplished primarily through phospholipid membranes by cells, a plethora of synthetic and biologic materials have been used to fashion artificial cell membranes with variable permeability, blood compatibility, surface properties, and moieties [4, 5]. For instance, McPhail et al. used a mixture of palmitol glycol chitosan and cholesterol to prepare an artificial outer membrane for their vesicle system [6], whereas, others have utilized biomaterials such as derivatized poly(lactide-co-glycolide) (PLGA) and derivatized poly(dimethysiloxane) to generate polymerosomes, with membrane-like compartmentalization capability [7–9]. A wide array of components can be incorporated into biomaterials-based compartments. These include live cells [10–12], enzyme systems [13–15], pharmacological drugs [16, 17], and antigens [18–20]. The number of biomedical applications is vast, ranging from red blood cell substitutes [21] to drug delivery systems enhancing targeted delivery to cells and organs [18].
These two broad biomimetic material strategies are also beginning to be explored in order to solve problems associated with immune cells, which is a wide-ranging field. For example, it has long been recognized that implantation of biomaterials triggers a profound reaction of host immune responses, collectively referred to as the foreign body reaction [22]. The physical injury due to implantation of biomaterials elicits an inflammatory response, considered to be part of the normal wound healing process. The presence of a biomaterial typically exacerbates this response, resulting in foreign body giant cell formation and antigen release (when a biological component is present) at the site of implantation [22]. Briefly, interaction with bodily fluid leads to protein adsorption on the surface of the biomaterial and can initiate the coagulation cascade, the complement system (which can polarize immune cells toward an inflammatory response), and the formation of a provisional matrix. These phenomenon have been extensively investigated on different biomaterial surfaces and it is thought that they are correlated to the physicochemical surface properties of the biomaterial, thereby linking biomaterial properties with host immune cell responses [23]. Following matrix formation, antigen-presenting cells, including macrophages and dendritic cells (DCs), can be recruited to the implant site by chemokines released by the matrix as well as surrounding cells. Macrophages, in particular, persist at the implantation site, adhering to the implant surface and coalescing with neighboring macrophages to form a giant cell body, which attempts to engulf the material. Within this encapsulation, macrophages secrete a number of inflammatory mediators, including reactive oxygen species and degradative enzymes that can be detrimental to the structure and functionality of the implanted biomaterial [23, 24].
More recently, biomedical engineers have taken aim at modulating host immune responses, including the foreign body reaction, to desired outcomes for improved diagnostic and therapeutic applications. This marriage of materials engineering and immunobiology has led to new immunomodulatory materials. This chapter highlights development and application of a number of immunomodulatory materials, categorized by the following general approaches: (a) surface motifs targeting cell surface receptors to direct immune cell responses; (b) morphogenic factor-related materials that release growth, differentiation, chemotactic, and immune-modulating factors; (c) stimuli-responsive materials that influence immune cell responses based on environmental conditions; and (d) self-assembly motifs, that when assembled, influence immune responses.
18.2 Surface Motifs
Surface modification with biomolecules is one of the most prevalent methods used to convey bioactivity to synthetic materials. There is a long history of literature establishing the influence of adsorbed adhesive proteins on cell adhesion, morphology, and migration at the material surface [25–27]. Adhesive proteins found naturally in the ECM, such as fibronectin, vitronectin, and laminin, provide multiple cues that direct diverse cellular processes via integrin binding [28–31]. Integrins, heterodimeric transmembrane proteins, are the key mediators between the ECM molecular signals and intracellular signaling pathways. On binding, integrins cluster and associate with various signal transduction molecules, thereby activating specific intracellular signaling cascades [25, 32–34].
The discovery of integrin-binding signaling domains, only several amino acids long, within ECM proteins prompted the development of material surfaces with ligated oligopeptide sequences. Short oligopeptide sequences have advantages over complete functional proteins, including their biospecificity, stability, lack of immunogenicity, and lower production/sterilization costs. Native ECM proteins tend to be immobilized on material surfaces in an uncontrolled fashion, such that presentation of specific domains is limited, as is selectivity for specific integrins and subsequent cellular interactions [2]. However, short adhesive peptides often have a relative decrease in activity due to loss of native conformation compared with the complete protein [35]. More recently, efforts have focused on the use of small protein fragments and conformationally constrained peptides, aiming to maintain biological activity while retaining the advantages of stability, lack of immunogenicity, and lower production cost [36–38].
To date, peptides containing the amino acid sequence arginine–glycine–aspartic acid (RGD), originally derived from fibronectin and found in numerous other adhesive proteins, have been the most extensively investigated as a bioadhesive motif [39, 40]. Other widely investigated ligands immobilized on material surfaces include tyr-ile-gly-ser-arg (YIGSR; derived from laminin), arg-glu-asp-val (REDV; derived from fibronectin), and val-pro-gly-ile-gly (VPGIG; derived from elastin) [41, 42]. Various materials (e.g., glass, quartz, metals, metal oxides, self-assembled monolayers, and polymers) have been used as model substrates for oligopeptide surface conjugation and the subsequent cellular responses on these surfaces were characterized [2, 39, 43, 44]. In vitro cell culture on biomimetic surfaces has resulted in enhanced cellular adhesion, spreading, focal contact, and cytoskeletal organization for several cell types including neurons [45], smooth muscle cells [46], endothelial cells [47], fibroblasts [38, 39], and osteoblasts [27, 48]. Although numerous investigations have clearly demonstrated (e.g., using RGD peptides) the difficulty in translating in vitro findings to in vivo scenarios [49, 50], application-specific successes have been demonstrated [51–53]. Nonfouling scaffolds, such as polyethylene glycol (PEG) hydrogels, thwart nonspecific cell adhesion and have been engineered to present various adhesive peptides [40]. The extent of integrin-mediated adhesion has been shown to be dependent on receptor–ligand affinity and on the density of the oligopeptide [42, 54–57]. For example, both cell migration and neurite extension have been correlated to adhesion strength, modulated in unimodal fashion by peptide density [58]. Through careful selection, it is possible to optimize for specific advantageous cellular response when designing scaffolds functionalized with adhesive ligands.
Biomaterial scaffolds engineered to present ECM proteins can also have a profound impact on host immune responses and have begun to be investigated, particularly for the attenuation of inflammatory responses. For instance, Acharya et al. demonstrated that culture of bone marrow-derived DCs on various ECM protein substrates (fibronectin, laminin, fibrinogen, serum, etc.) can result in differential levels of expression of proinflammatory surface molecules and pro- and anti-inflammatory cytokine secretion by DCs [28]. Historically, to modulate inflammatory responses to biomaterial implants, material scientists have manipulated material type, surface chemistry, and topography. These properties can influence protein deposition, provisional matrix formation, immune cell attachment, and ultimately, host immune responses [59]. Studies as those by Acharya et al. suggest that surface immobilization of naturally derived bioligands is an alternative approach with much promise for the mitigation of inflammatory responses to biomaterial implants [28]. Hume et al. adopted a similar approach, functionalizing PEG hydrogels with covalently immobilized immunosuppressive biological agents—transforming growth factor beta 1 (TGF-β1) and interleukin-10 (IL-10) to reduce inflammatory responses to cell-laden material carriers. Hume and coworkers immobilized TGF-β1 and IL-10 into PEG hydrogel networks via thiol–acrylate polymerization and showed that the presence of these two anti-inflammatory cytokines significantly downregulates DC maturation markers including IL-12 and MHC-II [60].
Functionalization of biomaterial surfaces with biologically derived molecules is also being applied to various immunotherapy approaches for cancer, infectious disease, autoimmune disease, and transplant rejection treatment. T cell adoptive immunotherapy, in particular, has emerged as a strategy with great potential for the treatment of a number of immune-related conditions. For instance, different T cell adoptive immunotherapies are now under clinical trial for treatment of human immunodeficiency virus (HIV), as well as different malignancies including breast carcinomas [61]. In this personalized medicine approach, patient-derived T cells specific for relevant antigens are isolated, engineered, expanded, and then reintroduced to the patient in an effort to amplify T cell responses to the malignant cell or infectious agent presenting the antigen [62, 63]. Conventionally, the ex vivo expansion of antigen-specific T cells is accomplished using autologous antigen-presenting cells, which have variability in phenotype and quantity. Additional drawbacks to the use of autologous antigen-presenting cells for T cell expansion include the time- and labor-intensive manufacturing process and its accompanying costs [64, 65]. As an alternative, material scientists have designed artificial antigen-presenting cells (aAPCs) as a solution to the use of autologous antigen-presenting cells. These acellular systems are typically based on polymeric microspheres [66], magnetic beads [67], or liposomes [68, 69]. For example, Tham et al. demonstrated that 5 μm polystyrene latex microparticles with surface immobilized B7-fusion proteins and a peptide–MHC complex can be an effective aAPC for in vitro antigen-specific T cell expansion [70].
Polymeric microspheres with surface-tethered immunoligands are also gaining widespread attention for their ability to manipulate DCs in DC-based immunotherapy, and more recently, for autoimmune applications. Lewis et al. reported that poly(d lactide-co-glycolide) microspheres with surface immobilized ligands (DEC205 and CD11c antibodies, and P-D2 peptide) are capable of enhanced DC targeting in vitro and in vivo without stimulating DC activation [71]. Similarly, Bandyopadhyay et al. demonstrated that DEC205-labeled PLGA nanoparticles not only efficiently target DCs for uptake but also increases production of IL-10 in DCs [72]. The findings from both of these studies could be instructive for the use of aAPCs to prevent and reverse autoimmune diseases. Along this line, researchers prevented and reversed diabetes in non-obese diabetic (NOD) mice using peptide–MHC complex coated iron oxide (FeO) nanoparticles. Santamaria and coworkers demonstrated that nanoparticles coated with disease-relevant peptide-major histocompatibility type I complexes (pMHC-I-nanoparticles) expanded cognate autoregulatory CD8+ T cells in animals, suppressed the recruitment of noncognate specificities, prevented disease in prediabetic mice, and restored normoglycemia in diabetic animals [73]. This study demonstrates the enormous potential of engineering surface motifs in biomaterials applications.
18.3 Morphogenic Factor-Related Materials
The development of synthetic materials able to sequester and deliver morphogenic/growth factors at the right place and right time is another established paradigm in biomimetic scaffolds for tissue engineering. Natural ECM has the capacity to bind not only large quantities of diverse growth factors and cell-inducing agents, but also to release these morphogens in a controlled manner, which results in coordinated protein expression, cell migration, proliferation, and differentiation. Proteoglycans are a subset of non-collagenous glycoproteins that contain glycosaminoglycan side chains. Examples include decorin, heparin, and chondroitin. The large reservoir of growth factors bound by proteoglycans is another vital feature of the ECM and is implicated in tissue remodeling, growth, and differentiation. This function of the ECM is crucial for neotissue formation and repair [3, 74]. Synthetic analogs have replicated growth factor activity by regulating their display, their release kinetics, and their biostability. Sakiyama-Elbert et al. demonstrated that cell-mediated release of heparin-binding growth factors from fibrin scaffolds with immobilized heparin can enhance neurite extension [75]. This model capitalizes on the affinity of heparin for growth factors such as transforming growth factor beta (TGF-β) and fibroblast growth factor (FGF), controlling their presentation and delivery rate [76].
A more common delivery approach involves the sustained, controlled release of soluble morphogenic factor, previously dispersed into the matrix of the scaffold during preparation. This approach affords sustained, localized delivery of tissue inductive factors in a spatiotemporal manner to direct specific tissue responses as well as minimize off-target effects typically associated with systemic delivery. A wide array of matrices and scaffolds has been used to accommodate delivery of drugs that regulate multiple processes of cell chemotaxis, attachment, proliferation, differentiation, and morphogenesis [25, 77–79]. For instance, Lucas et al. showed that the incorporation of a water-soluble bone morphogenetic protein (BMP) extract from bone matrix into a synthetic polyanhydride matrix promoted chondrogenesis and osteogenesis when implanted ectopically in vivo [80]. Notably, this study demonstrated that only in combination are the polyanhydride matrix and osteogenic protein extract able to effect cartilage and bone formation. Additionally, their results highlighted the short half-life of proteins in vivo. Pharmaceutical scientists have developed ways to avoid this drawback such as PEGylation, which through the covalent linkage of PEG, stabilizes proteins, helps them resist protein adsorption and aggregation, and reduces their uptake by cells and therefore, immune system intervention [81, 82]. Alternatively, approaches utilizing the protein-making machinery of cells have been explored. Gene transfection is a potent and promising technique that involves the in vitro or in vivo incorporation of genetic material such as DNA, RNA, and RNAi [83–86] into cells for experimental and therapeutic purposes. Gene delivery provides for sustained therapeutic levels of protein and targeting of multiple cellular processes. Moreover, several investigators have reported successful tissue regeneration by gene transfection of tissue-building cells [87, 88]. However, there are immunological and safety concerns associated with viral vectors used in these studies, motivating development of nonviral vector systems [59].
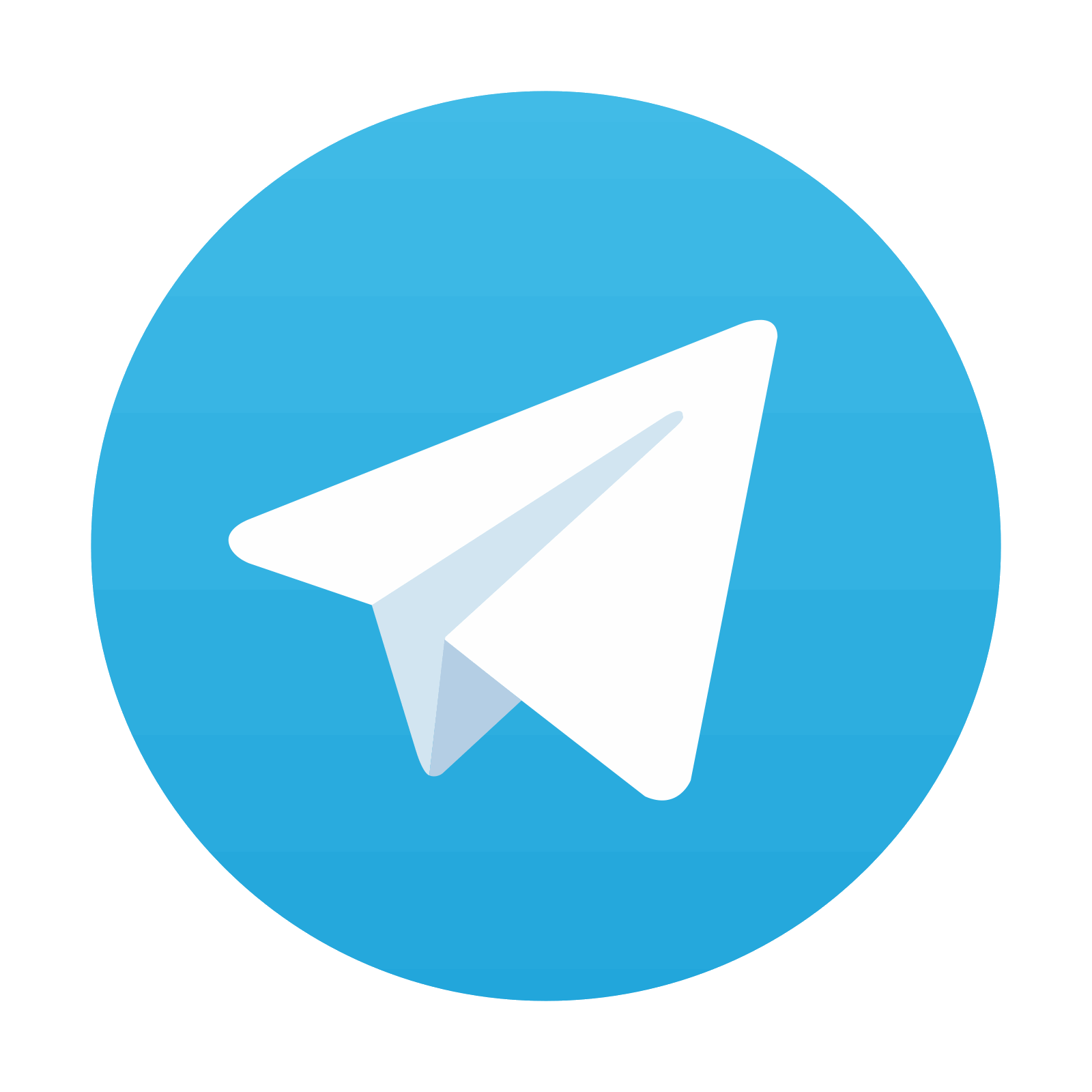
Stay updated, free articles. Join our Telegram channel
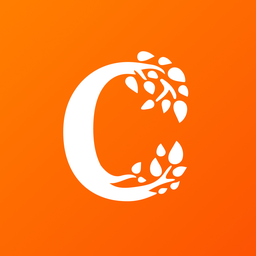
Full access? Get Clinical Tree
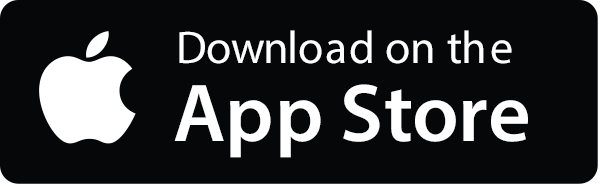
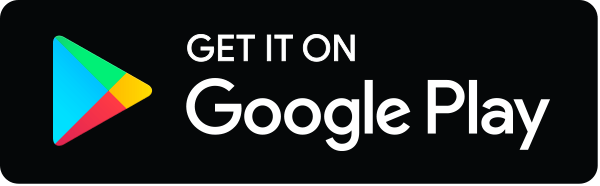