Engineering Immune Responses to Allografts
Diabetes Research Institute, Leonard M. Miller School of Medicine, University of Miami, Miami, FL, USA; Department of Biomedical Engineering, College of Engineering, University of Miami, Coral Gables, FL, USA
Diabetes Research Institute, Department of Surgery, Leonard M. Miller School of Medicine, University of Miami, Miami, FL, USA; Department of Biomedical Engineering, College of Engineering, University of Miami, Coral Gables, FL, USA
17.1 Introduction
Tissue engineering, the combination of cells, biomaterials, and bioactive factors to repair, replace, or maintain a dysfunctional tissue, has tremendous potential in the treatment of numerous pathological conditions. The source of the cellular components within the tissue-engineered implant is a critical issue in clinical translation, whereby disparate sources, that is, autologous, allogeneic, or xenogeneic, are met with distinct challenges. Allogeneic third-party cells or tissues have the highest marketing and translational potential in that they may be banked or precultured for alacrity of use. Furthermore, they have the advantage over autologous, self-sourced cells, in that they avoid secondary trauma due to isolation and are of more consistent quality, as often the desired cells of the patient are damaged, aged, or limited. Allograft transplantation, however, is restricted by the need for systemic immunosuppressive drugs to prevent rejection of the foreign cells. While xenogeneic sources have large appeal due to their availability, viral transmission risks and limits on phenotypic compatibility restrict their widespread use. As such, when the cell source is either limited or dysfunctional in the patient, for example, organ failure, allogeneic transplantation is currently the only clinically relevant option.
Whole organ allogeneic transplantation is the most common type of allograft, beginning with the first clinical kidney transplant in 1956 [1]. Currently, over 25,000 whole organ transplants are performed each year in the United States, with 15,152 kidneys and 5527 livers transplanted in 2012 alone [2]. The field of allotransplantation has expanded to encompass transplanted tissues, such as skin grafts, cells, bone marrow, or islets of Langerhans. In the future, it is envisioned that engineered tissues comprised of allogeneic cells will make up a large proportion of future transplants customized to fit the needs of the patient.
One of the biggest challenges to the clinical success of allogeneic transplants is the interaction between the host immune system and the foreign transplanted cells. Before delving into the unique response of the immune system to allografts, one should first understand the basic components of the immune system and its function under homeostasis (for a more extensive review, see Reference 3). The human immune system consists of the innate and adaptive immune systems, in which each branch plays a particular role in combating disease and infection.
17.1.1 Key Components and Pathways of the Immune System
The innate immune system provides intrinsic protection against microbes and consists of epithelia, phagocytes, natural killer (NK) cells, cytokines, and the complement system. Epithelia provide the physical barrier to pathogens entering tissues. Phagocytes are cells that ingest and break down microbes and include neutrophils, monocytes, and macrophages (MΦ). The primary role of NK cells is to eradicate microbe-infected host cells and release interferon-gamma (IFN-γ), a cytokine that directs MΦ to lyse their phaged substances. Last, the complement system consists of a family of proteins whose roles include: coating microbes to tag them for phagocytosis, and forming pore-inducing membrane complexes to induce microbe lysis. The recognition of pathogens occurs via molecular pattern recognition, in which pathogen-recognition receptors (PRRs), encoded in the germline, recognize generalized patterns expressed by infected cells. These patterns include damage-associated molecular patterns (DAMPs) and common molecules conservatively expressed on microbes, such as pathogen-associated molecular patterns (PAMPs). Although it is based on receptors to sequences found ubiquitously on microbes or stressed cells and is limited in adaptation, the innate immune system is still highly targeted, potent, and evolving. Perhaps the most well-known function of the innate immune system is instigating inflammation.
Acute inflammation is typically the primary response to any foreign invasion or trauma. Neutrophils are the dominant first responders, which initiate phagocytosis of microbes and release cytotoxic signals to degrade damaged cell components. The neutrophil response is followed by monocyte migration to the site. Without prompt resolution, the acute phase transitions to chronic inflammation, hallmarked by the prolonged presence of monocyte-derived MΦ. Chronic inflammation inevitably leads to damage of the native tissue. If the phagocytes are unable to phage the foreign substance, MΦ will fuse into clusters known as foreign body giant cells, large multi-nuclear cells characteristic of chronic inflammation. Ideally, chronic inflammation will be avoided in an allograft and the immune response can resolve as granulation tissue.
The adaptive immune system consists of cell-mediated immunity, primarily carried out by T lymphocytes, and humoral immunity, which involves antibodies generated by B lymphocytes. Contrary to the innate immune system, adaptive immunity is highly specific, as receptors expressed by a particular T or B cell clone only recognize a specific antigen. When the receptors of a particular clone bind antigen, specific clonal expansion occurs, permitting an aggressive and targeted attack of specific pathogens. Additionally, immunological memory facilitates enhanced protection following initial exposure to a particular antigen. Prior to antigen activation, T and B cells are considered naïve. Following activation, these lymphocytes are termed effector cells.
Cell-mediated immunity is conducted by T cells, which contain the cluster of differentiation (CD) marker CD3. These cells mature in the thymus to their distinct phenotype, the primary types being CD8+ cytotoxic T (Tc) cells and CD4+ helper T (Th) cells. Tc cells bind to antigen presented by major histocompatibility complex (MHC) I receptors. Tc cells are subsequently activated when costimulated with cell-surface proteins CD80 or CD86, which complex with the CD28 receptor on the T cell. On activation, Tc cells are capable of directly killing the infected cell by releasing perforins, which create pores in the membrane of invading cells and by releasing apoptotic factors inducing granzymes. Th cells are activated on encountering antigens presented by MHC II receptors on professional antigen-presenting cells (APCs). APCs include dendritic cells (DCs) and MΦ, which either reside in the peripheral tissue or are recruited to the site via chemotactic gradients. APCs present antigen to Th cells, typically in the lymph node, whereby activated Th cells secrete cytokines and assist other cells involved in the adaptive immune response, such as Tc, MΦ, and B cells. Each subtype of effector Th cells, for example Th1 or Th2, releases a signature cocktail of cytokines that leads to a characteristic immune response. Another subtype of CD4+ Th cell is the CD25+FoxP3+ regulatory T cell (Treg), which facilitates downregulation of the immune response following elimination of the antigen. Tregs instruct effector T cells via immunosuppressive cytokines, such as transforming growth factor beta 1 (TGF-β1), interleukin-10 (IL-10), and IL-35, and/or cell surface receptors such as galectin-1 [4]. Furthermore, Tregs interact with and suppress the function of APCs through a number of mechanisms, including cytotoxic T lymphocyte antigen 4 (CTLA-4), a Treg cell surface receptor which results in decreased costimulation by APCs [5].
In humoral immunity, B cells and antibodies, that is, immunoglobulins (Igs), are the primary players. B cells are first generated in the bone marrow and then they undergo migration to the secondary lymphoid tissues. B cells are able to recognize antigens directly without MHC-restricted presentation by infected cells or APCs via their B cell receptor (BCR), which is an immobilized antibody molecule (IgM) specific to a particular B cell clone. Following the binding of the BCR by complementary antigen and with support from Th cells, B cells differentiate into either effector plasma B cells or memory B cells. Effector plasma B cells secrete Ig, primarily IgG, for humoral targeting of microbes, tagging them for phagocytic destruction. Meanwhile, memory B cells remain in the body following antigen exposure to facilitate a more robust response if reinfection occurs.
Components of the immune system must undergo self-selection to learn which is a friend and which is a foe. Central tolerance for self-antigens is achieved via clonal selection, which occurs in the bone marrow for B cells and the thymus for T cells. Autoimmune diseases, such as rheumatoid arthritis, multiple sclerosis, and diabetes, occur when the immune system fails to tolerate itself and mounts an antigen-specific attack of host cells and/or tissues. Under normal conditions, lymphocyte clones with B and T cell receptors expressing strong affinity for self-antigens undergo negative selection and are targeted for apoptosis. In autoimmunity, this process of negative selection goes awry, and self-reactive lymphocytes survive and attack native tissue. Often, infections can play a role in instigating autoimmune disorders, as they can cause the presentation of self-antigen to self-reactive immune cells to occur with a costimulatory signal [6].
17.1.2 Immune Response to an Allograft
Once the components of the immune system and their standard response to antigen are understood, one can examine the immune response in the context of an allograft transplant. On transplantation of cells with or without a material, the initial response will be acute inflammation, whereby resident APCs respond to the transplant and monocytes are directed to the site. For allogeneic cells, the host recognizes some of the surface proteins on transplanted cells or released cellular components as nonnative, thus treating them as antigens. The principal antigens recognized are MHC I and II. Every human expresses six MHC I and six MHC II alleles, each of which contains hundreds of subtypes, resulting in transplanted cells expressing proteins recognized as foreign to the host. The host may recognize alloantigens via two pathways, as shown in Figure 17.1: direct antigen presentation or indirect antigen presentation. Direct antigen presentation is where a T cell, specific for the alloantigen, contacts the specific surface proteins of the transplanted cell. If the foreign cell is an APC, then CD4+ T helper cells can be activated. If the foreign cell is not a professional APC, then CD8+ T cytolytic cells are activated. While it was previously thought that CD4+ T cells were the primary players in alloantigen recognition, it is now known that both CD4+ and CD8+ T cells play critical roles [7]. For indirect activation, graft rejection is initiated by alloantigens released by the allograft, the most potent being alloantigens released during cellular necrosis, which are coreleased with DAMPs [8]. DCs and other APCs in the periphery can phage these alloantigens, migrate to the lymphatics, and subsequently present antigen to generate clonally specific effector T cells. Alloantigens present in the interstitium can also undergo transport through the lymph, where they can bind to complementary B cells or be phaged by residing APCs to be presented to complementary T cells. Depending on the local cytokine environment which alters APC costimulatory receptors and signaling pathways, the resulting T cell response can vary from a characteristic Th1, Th2, Th17, or regulatory response, as defined by the phenotype of the prevalent CD4+ T cells [9]. Th1 CD4+ cells are the primary players in causing allograft rejection, through the production of the cytokine IL-2, which leads to the proliferation of effector CD8+ cytolytic T cells, although Th2 and Th17 responses also play a role in mediating transplant rejection [10]. Occasionally, immune senescence occurs, where the host immune system is still primed to react against the allograft antigens, but constant exposure and immune challenges lead to the immune system reducing its attack, creating a symbiosis between the transplant and the host tissue [11]. Overall, however, the standard immune response results in allograft rejection, involving alloantigen uptake by APCs, presentation of antigen to generate effector Th cells and alloantibody producing B cells, and culminating in cytolytic T cells inducing cell death of the transplanted cells via direct cell–cell contact.

Occasionally, specialized circumstances cause additional immune problems with allografts, such as cases of graft-versus-host disease (GVHD). In these transplants, the host rejects not only the graft, but donor immune cells present in the graft recognize the host as foreign and begin to attack the host, generating a dangerous and potentially life-threatening situation [12]. The transplantation of a cell type in which the original native cells were destroyed via autoimmune responses represents another unique immunological condition. For instance, allogeneic islets are transplanted in the treatment of type 1 diabetes [13], an autoimmune disorder that results in select immunological destruction of beta cells within the pancreatic islets of Langerhans. The resulting immunological response is particularly aggravated, as the recipient is primed with autoreactive T and B cells specifically targeting the type of cells being transplanted, in addition to the standard response targeting alloantigens.
17.1.3 Current Approaches to Mitigating Allograft Rejection
Once an allograft has been implanted, there are two main goals. The first is to achieve adequate function, and the second is to maintain function, which requires immune acceptance of the graft. Currently, allograft recipients rely on the administration of systemic immunosuppressant or antirejection drugs. The use of these agents is required to prevent, or at least dampen, T and B cell activation to the foreign antigens. Systemic immunosuppression typically involves two stages, an induction stage, followed by a maintenance regimen. For kidney transplants, the most common induction routine is a combination of a CD3+ T cell depleting regimen (e.g., Thymoglobulin, Genzyme Corp., Cambridge, MA), followed by administration of anti-IL-2 receptor (e.g., basiliximab), which prevents IL-2 signaling and subsequent lymphocyte proliferation. For maintenance, a calcineurin inhibitor (e.g., tacrolimus) and a mechanistic target of rapamycin (mTOR) inhibitor (e.g., sirolimus), which block IL-2 secretion and responses, respectively, are used in combination with an anti-inflammatory glucocorticoid (e.g., prednisone) [14]. While protocols vary based on individual patient circumstances and the type of allograft performed, the goal is to dampen overall immune activation, resulting in systemic suppression of the immune system. As such, the general use of systemic immunosuppressants has a number of crucial disadvantages, such as increased susceptibility to disease, infection, and cancer [15]. Other side effects include increased risk of cardiovascular disease, hypertension, hyperlipidemia, and new-onset diabetes [16]. Transplant patients are resigned to a heavy drug regimen for the duration of their life, a difficult challenge requiring continual patient diligence. Furthermore, the financial burden of lifelong antirejection therapy is substantial, costing several thousands of dollars annually. For example, the antiproliferative agent mycophenolatemofetil, commonly prescribed for organ transplants, has an average wholesale price of $14,921 for an annual supply of the branded product and $11,578 for the generic [17]. Finally, even with long-term use of immunosuppressive therapy, a smoldering, chronic graft rejection typically occurs, leading to progressive decline of graft function [18].
Due to the weighty disadvantages associated with systemic immunosuppression, researchers are developing alternative treatment options, with a focus on generating a graft microenvironment that favors immunological tolerance. Immunological or operational tolerance is defined as a lack of reactivity to donor alloantigens in the absence of long-term immunosuppression, while retaining the capacity of the immune system to react to other foreign antigens or insults [19]. Immunological tolerance is a dynamic state, in which the plasticity of immune cells results in the ability to induce tolerance or activation. Thus, the success of protocols designed to induce tolerance has been unpredictable. However, a recent focus on engineering a tolerogenic microenvironment at the allograft transplant site may prove to be more successful, as peripheral tolerance is induced locally.
17.2 Engineering Strategies forImmune Acceptance
Immune acceptance of an allograft can be engineered using a number of complementary approaches, with each approach seeking to generate a graft site conducive to long-term acceptance. One approach is changing the anatomical location of the graft, an adjustable parameter when transplanting cells and smaller tissues. Variations in resident inflammatory or tolerogenic cells, as well as the degree of immunological access, can greatly affect the severity of the initial immunological response to the transplant, with some areas deemed immune privileged. Alternatively, the use of methods to camouflage transplanted cells from the native immune system and prevent antigen recognition is common. These cloaking strategies include encapsulation and the grafting of cell surfaces with benign polymers. Another approach is the creation of a protective and immune-tolerant zone surrounding the allograft to provide instruction to nearby immune cells. These tolerogenic microenvironments can be achieved through the cotransplantation of cells with immunosuppressive properties, the delivery of immunomodulatory drugs or cytokines, and/or the generation of biomimetic surfaces capable of inducing immunoregulatory pathways, as summarized in Figure 17.2.

17.2.1 Selection of Allograft Site
Select areas of the body are deemed immune privileged, making them attractive transplant sites for allografts. These sites are identified by a decreased immunological response to antigen exposure. As such, these sites exhibit enhanced allograft survival when compared with the typical rejection profile observed elsewhere in the body, although the use of these sites does not prevent of graft rejection. In general, immune-privileged sites are natively endowed with unique characteristics, such as a blood barrier, the expression of molecules that actively inhibit immune cells, the increased presence of regulatory immune cells, and a generalized decrease in MHC I expression (for a cohesive review, see Reference 20). Tissues that have expressed this privilege include the eye, testis, brain, and pregnant uterus [21].
Immune privilege in the eye was first noted by the observation that corneal allografts fared better than skin allografts in similarly mismatched patients. The eye contains a blood–aqueous barrier and a blood–retinal barrier consisting of tight junctions between epithelial cells, which not only functions to help maintain intraocular pressure, but also separates the blood and lymphatic systems from the eye. Selected tissues within the eye contain cells that release mediating cytokines, such as the myeloid cells in the retina [22]. Retinal pigment epithelial (RPE) cells are known to express coinhibitory molecules, such as Fas ligand (FasL), programmed death 1 ligand 1(PD-L1), and CTLA-4, which suppress effector T cells [23]. Furthermore, parenchymal cells of the eye have been shown to be capable of inducing Tregs that suppress CD4+ Th1 effector cells [24]. With this dampened immunological activity, the eye has been explored as an allograft implantation site. In a study by Hatchell et al., pancreatic islet allografts were delivered to the subretinal space of cat eyes, where the islets survived without rejection for up to 3 weeks [25]. The risk of damaging the eyesight, however, restricts the use of this site for cell transplantation. To avoid this concern, selected immunoregulatory cells found within the eye have been exploited to induce tolerance, such as the combination of retinal progenitor cells and biodegradable polymers to engineer an immunoprivileged site [26]. Resulting grafts, transplanted in subrenal capsules of allograft recipients, demonstrated enhanced tolerance.
The testis, which is exposed to a number of unique proteins during sperm production, maintains an immune-privileged environment in order to protect the process of spermatogenesis from immune attack. This privilege has been observed through the resistance to rejection of allograft testes, ectopically transplanted under the skin of Balb/c mice without systemic immunosuppression [27]. Similar to the eye, the testis contain a number of compounding factors that contribute to immune privilege, such as a blood–testis barrier, a decrease in inflammatory MΦ, increased numbers of Tregs, and a lack of resident B cells [21]. The transplantation of cells and tissues to this site has shown increased survival in the absence of immunosuppression, such as the transplantation of islets [28], parathyroid, and skin [29]. The delay in allograft rejection at this site, however, has been modest and limited to small animal models [30]. Alternatively, the isolation and cotransplantation of selected cells found in the testis, notably Sertoli cells, have fared better. The cotransplantation of Sertoli cells and various other allogeneic cells with allografts have demonstrated beneficial effects, as highlighted in later sections.
The benefits of transplanting into an immune-privileged site must be weighed against the optimal site for graft efficacy and function. For example, pancreatic islets, used for the treatment of type 1 diabetes, require adequate vascularization to achieve optimal oxygenation and glucose responsiveness [31]. At these immune-privileged sites, the blood barrier that imparts immune protection may impede adequate cellular engraftment. As a result, the use of immunoprivileged sites for allograft transplants remains a promising avenue worthy of exploration, but this approach is not the silver bullet of engineering graft immune acceptance. Furthermore, the designation of immune privilege is a misnomer as an immune response is still typically observed, particularly when the blood barrier is breeched during transplantation. For example, the transplantation of fully mismatched allograft islets into the eye resulted in a significant T cell infiltrate surrounding the graft site at 14-days posttransplantation. While full graft rejection was slightly delayed compared with transplantation into the kidney capsule (average rejection time of 30 days vs. 15 days, respectively), full allograft rejection invariably occurred [32].
17.2.2 Cellular Encapsulation
The use of biomaterials to encapsulate cells accomplishes a key set of desired outcomes. In tissue engineering, the presence of a biomaterial gives cells a substrate to attach to and grow on, acting as a scaffold. Scaffolds provide mechanical support for implanted cells to proliferate, deposit extracellular matrix, and integrate with the native tissue. In the context of allografts, cells can be seeded within materials, not only for support, but also for masking immune recognition. Although the complexity and robustness of the immune system makes camouflaging foreign antigens through material encapsulation challenging, strategies are being developed to enhance the effectiveness of these engineered immunological barriers while still allowing for healthy allograft function.
While allogeneic cells can be encapsulated into various geometries, from sheets to rods to discs, the most common geometry employed are spheres (typically 600–1000 μm in diameter), given their high surface to volume ratio and ease of transplantation [33]. When selecting biomaterials for encapsulation, desired properties of materials include biocompatibility, cytocompatibility, mild scaffold production conditions, and biostability. Biocompatibility is essential for encapsulation, as even a mild foreign body response (FBR) to the coating can lead to significant decreases in nutrient delivery to the embedded cells. Cytocompatibility and mild encapsulation conditions are critical to ensure that the process of material encapsulation does not impart damage to the allogeneic cells; cell damage and necrosis leads to the release of danger signals and cellular lysis products into the local graft microenvironment, instigating inflammatory processes and increasing alloantigen dumping. The importance of biostability of the resulting coating is clear, as the duration of immunoprotection must be long-term. Commonly used materials for encapsulation include alginate, agarose, and polyethylene glycol (PEG) [34]. These materials are fabricated into hydrogels, which are water-containing hydrophilic polymers supported by physical or chemical cross-links. Biomaterials fashioned into hydrogels are highly functional in allowing encapsulated cells to perform their roles, for example, permitting appropriate levels of nutrient and the secretion of agents to and from the cells while simultaneously acting as a barrier to host cell to donor cell interactions [35].
Alginates are polysaccharide biopolymers of D-mannuronic acid and L-guluronic acid residues and are often used for the encapsulation of cells. Isolated from seaweed, their attractive properties include their biocompatibility, their nonimmunogenicity, and their ability to form hydrogels with instant gelation using multivalent cations. As cells do not adhere to alginate naturally, this material can be modified with cellular adhesion proteins to facilitate cell attachment [36]. While not inherently degradable, the ionic gelation process does result in gels that weaken over time due to ion exchange [37]. As such, efforts to stabilize the resulting capsule have been explored, such as the incorporation of covalent cross-links within the hydrogel [38]. For example, the functionalization of alginate with alkyl azide, followed by the addition of PEG cross-linkers functionalized with 1-methyl-2-diphenyl-phosphino-terephthalate (MDT), results in the formation of amide bonds via Staudinger ligation, a spontaneous, bioorthogonal reaction between an azide and phosphine known for its benignity. This approach demonstrated increased mechanical stabilization of the alginate, with stable hydrogels even following the removal of ionic cross-links [39]. Cytocompatibility of the cross-linked gels was high, with no adverse effects on viability or function observed for both beta cells and islets [40].
Evaluation of alginate-based capsules for immunoprotection of allografts in rodent models has been extensive, thus, complete review of all promising approaches would be lengthy [41]. In all, multiple groups have demonstrated long-term protection of alginate-based capsules within rodent allogeneic models, both for chemically induced and spontaneous diabetic models. Notably, barium cross-linked, islet loaded, alginate capsules, transplanted into syngeneic nonobese diabetic (NOD) mice, have shown extended protection, with graft function demonstrated over 350 days [42]. In larger animal models, published reports are limited, with most evaluating porcine islets and function observed when combined with immunosuppressive agents [43]. One study of particular interest, however, transplanted alginate encapsulated porcine islets into nonimmunosuppressed primates, with islet survival and function up to 6 months posttransplantation [44]. Of note, only chemical-induced diabetic models are available for nonhuman primate, thus autoimmune effects cannot be evaluated at this time in these animal models.
Agarose, a neutral polysaccharide, is biologically inert and does not adsorb proteins or cells, making it an attractive candidate for use in cellular encapsulation. The mechanical properties of agarose can be tailored by varying its concentration, and the material undergoes gelation on exposure to reduced temperatures [45]. Agarose has been used for immunoisolation in geometries ranging from slabs to microbeads, although controlling the size of the resulting microbeads is challenging. Agarose gels have demonstrated durability in vivo. For example, islet loaded 5% agarose microbeads remained intact 400 days posttransplantation, with functional islets and no immune cell infiltration in a mouse model of autoimmune diabetes [46].
PEG is a biocompatible molecule often used for encapsulation. PEG is highly inert, with flexibility in molecular weights and geometries from unbranched to multiarm configurations. PEG is easily tunable via functionalization of end groups, resulting in a wide variation of mechanical, chemical, and bioactive properties [47]. Commonly, photopolymerization has been utilized to achieve gelation; however, care must be taken in the duration and concentration of agents to minimize formation of free radicals that can damage encapsulated cells [48]. In an alternative approach, Teramura et al. utilized PEG hydrogels functionalized with maleimide or thiol to microencapsulate mouse islets. Ligation between the maleimide and thiol groups occurs efficiently, resulting in covalently cross-linked microsized coatings [49]. This microcapsule formulation was shown to retain islet function and reduce immune complement activation compared with controls; however, the coating uniformity and thickness was unpredictable. An additional facet of PEG is the use of the polymeric backbone to incorporate bioactive molecules and motifs. Lin et al. utilized this tunability to incorporate beta cell adhesion receptor EphA5 and ligand ephrinA5 within hydrogels, resulting in decreased anoikis of the cells following encapsulation [50].
The clinical implementation of encapsulation has the potential to treat numerous conditions, from diabetes to Parkinson’s to myocardial infarction, whereby the allogenic cells, embedded within the polymer, can respond to host cues and secrete desired factors. Progress in the clinical area has primarily been in the field of islet transplantation to treat type 1 diabetes, although success has been limited [51]. In a clinical trial taking place in Italy, four patients implanted with islet intraperitoneal allografts microencapsulated in sodium alginate were examined for their immune response to the allograft up to 5 years posttransplantation [52]. These patients did not receive systemic immunosuppression and no directed immune response was found to the encapsulated islets, measured by the lack of antibodies to MHC and islets. Living Cell Technologies (LCT) has also performed clinical islet transplantation of encapsulated porcine islets using porcine islets sourced from specialized herds. In these limited trials, LCT has claimed a marked decrease in hypoglycemic episodes in these patients [53]. Clinical outcomes of encapsulated islets, from either allo- or xeno- sources, have yet to result in long-term independence from exogenous insulin.
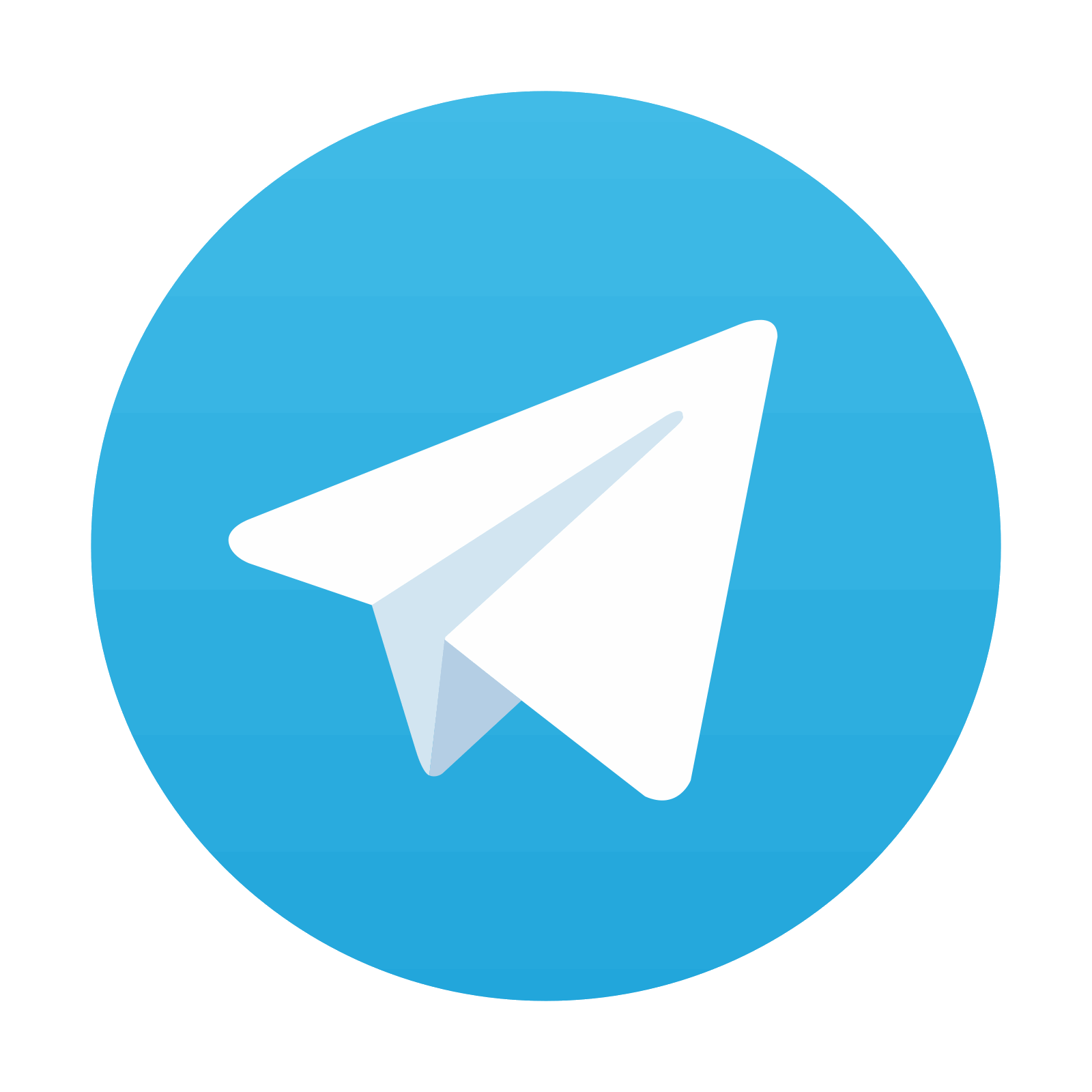
Stay updated, free articles. Join our Telegram channel
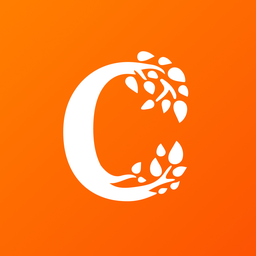
Full access? Get Clinical Tree
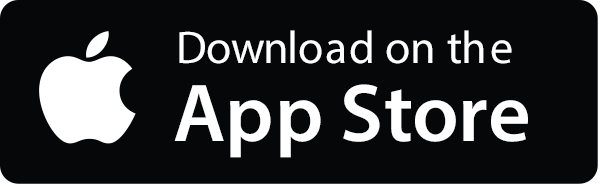
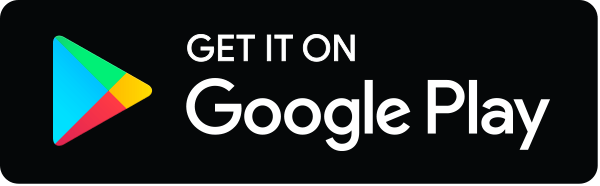