Learning from Tissue Equivalents: Biomechanics and Mechanobiology
Department of Biomedical Engineering, Yale University, New Haven, CT, USA
15.1 Introduction
Advances in tissue engineering and regenerative medicine have been tremendous over the past decade. Clinical successes include a number of implantable tissues, with the majority of available products being in orthopedic and wound healing arenas [1]. Still, a major challenge in research and development is optimization, both in the performance of the tissue-engineered construct and in the scale-up of processes required for commercialization.
Tissue engineering approaches also benefit basic research in biomechanics and mechanobiology. Tissue equivalents represent excellent model systems for studying cellular responses to mechanical and chemical stimuli, both of which can be well controlled in vitro. Whereas biomechanics seeks to understand bulk material behavior in terms of microstructure, which can be manipulated in a tissue-engineered construct, mechanobiology seeks to correlate cellular responses with mechanical stimuli, which is often difficult to infer in vivo and even in native tissues in vitro. Mechanically stimulated cells can alter their local environment, often by working on and remodeling the local extracellular matrix (ECM) and hence, bulk material behavior. Such changes, in turn, can change the mechanical stimuli sensed by the cell and thereby lead to additional cell-mediated changes of the construct.
A combined approach using tissue equivalent-based experiments and computational models of cell–matrix mechanics promises to provide increased insight into these complex couplings. Quantitative measurement of cellular gene expression and protein synthesis can be correlated with mechanical stimuli via mechanical testing and characterization of tissue equivalents. This information can then inform computational simulations to correlate how the mechanical stimuli and subsequent cellular responses lead to matrix remodeling.
In this chapter, we review prior observations of three types of tissue equivalents (free-floating, uniaxially constrained, and biaxially constrained; see Figure 15.1), prior work in the mechanical characterization of the tissue equivalents, and prior theoretical work on mathematically modeling the growth and remodeling (G&R) of tissue equivalents. Finally, we propose a possible methodology for combining experiments and computational simulations to examine cell-mediated remodeling of tissue equivalents.

15.2 Background
15.2.1 Matrix Composition and Integrins
The composition of the ECM varies depending on tissue type, in vivo location, and tissue health. Most components of the ECM are proteins, with collagen often being the most prevalent. Collagens consist of large domains of the repeating peptide sequence Gly-X-Y that are folded into a triple-helix structure, with X and Y representing different amino acids, often proline or hydroxyproline. The collagens are divided into subfamilies, mainly fibrillar (e.g., type I, II, and III) and network-like (e.g., type IV), with most of the other collagen types playing accessory roles for the two dominant subfamilies [2]. Of the fibrillar collagens, type I is found throughout the body and is a substantial structural component in tissues such as skin, arteries, tendons, and ligaments. Type II collagen is found primarily in cartilage, whereas type III collagen is often associated with type I, except during early wound healing when it precedes type I. During the formation of collagen fibers, lysyl oxidases convert some of the hydroxylysine and lysine residues into aldehydes, which allows the formation of covalent cross-links between fibrils and aids in the aggregation of collagen fibers [3]. Collagen is continually turned over by cells (i.e., degraded, removed, and replaced), which allows tissue to adapt to changes in both the mechanical and chemical environment [4].
Other common ECM components include elastic fibers, adhesion molecules, and proteoglycans (PGs), which consist of a protein core and associated glycosaminoglycans (GAGs). Elastic fibers consist primarily of elastin, but also associated glycoproteins such as the fibrillins and fibulins; elastic fibers endow tissues with resilience, recoil, and greater degrees of extensibility than does collagen alone. Elastin tends to be produced primarily during the perinatal period; hence, it has a minimal role in tissue adaptation and wound repair [5]. Among the many adhesion molecules, fibronectin is a glycoprotein that is important in the migration and localization of many cell types due to its repeated arginine–glycine–aspartic acid (RGD) domains, which serve as ligands for multiple cell–matrix adhesion receptors. Fibronectin can also bind a variety of growth factors that can contribute to cellular differentiation [5]. PGs and GAGs play diverse roles in tissue maintenance and adaptation. They are important in the assembly or organization of other matrix components, including collagen, through the creation of a swelling pressure due to abundant fixed negative charges. By sequestering water, they help resist compressive loads, which is particularly important in tissues such as cartilage. PGs and GAGs can also sequester growth factors and thereby modulate cell behavior [6].
Cell–matrix interactions are important in mechanical sensing by cells and the regulation of cellular gene expression and protein synthesis, which together enable physical remodeling of the matrix. The primary receptors responsible for cell attachment to the ECM are the integrins. These heterodimeric structures consist of one α and one β subunit, each of which spans the plasma membrane of cells. The extracellular domain binds to ECM molecules and the intracellular domain is anchored to the cytoskeleton. This association with the cytoskeleton allows the transduction of mechanical stimuli (outside-in signaling) as well as the transmission of actomyosin-based forces to the matrix (inside-out signaling), and can thus affect multiple signaling pathways [7]. The α and β subunit pairing determines the ligands with which the integrin will associate. For example, αv-based integrins associate with the RGD peptide found in fibronectin and vitronectin, while α1β1 and α2β1 associate with collagen through the GFOGER peptide sequence.
15.2.2 Fibroblasts
The cell type often associated with connective tissue development, maintenance, and remodeling is the fibroblast. Fibroblasts are found in many tissues of the body and can display different cellular behaviors depending on embryonic origin, surrounding matrix, and biochemical cues [8]. Fibroblasts can produce a wide range of ECM proteins, including collagens, fibronectin, and a wide array of PGs and GAGs [9]. Fibroblasts can also differentiate into the myofibroblast phenotype, which is often indicated by the cellular expression of alpha smooth muscle actin, denoted α-SMA [10]. This phenotypic change to a myofibroblast appears to require a tensile mechanical environment and cell exposure to transforming growth factor beta (TGF-β1, one of three primary isoforms); it increases the magnitude of force the cell can apply to the surrounding matrix [11].
15.2.3 Growth Factors and Culture Media
The terms cytokine and growth factor are often used interchangeably. These biomolecules are soluble proteins or glycoproteins that are secreted by cells and act nonenzymatically to regulate cellular functions via both paracrine and autocrine stimulation [12]. Functions regulated by growth factors can be diverse, and a single growth factor can elicit opposite effects (e.g., proliferation or apoptosis) in the same cell type due to differences in other cellular cues like the matrix environment or the presence of other growth factors. Two growth factors of importance for fibroblasts and collagen maintenance are platelet-derived growth factor (PDGF) and TGF-β1. PDGF can act as a chemotactic agent, which is important for wound healing to induce fibroblasts and other cell types to infiltrate the wound site. Furthermore, PDGF can induce proliferation, and it can stimulate the production of matrix components and matrix metalloproteinases (MMPs) that contribute to the degradation of many ECM proteins. Hence, PDGF can play an important role in tissue maintenance and remodeling [13]. TGF-β1 has diverse effects on cells that depend strongly on other environmental factors. It has been shown, for example, to stimulate both proliferation and apoptosis, to be required for cell phenotypic modulation, and to increase matrix deposition while downregulating MMPs. In terms of cellular activity, TGF-β1 “seems capable of doing just about everything” [14]. Other cytokines of importance include the interleukins (e.g., IL-2 or IL-6). They are produced by leukocytes and often play important roles in vivo in tissue remodeling, wound healing, and disease progression.
Cell culture media for in vitro cell and tissue experiments is necessary to ensure the viability of resident cells, as well as to serve as a source of molecules needed for matrix production and other cell-mediated processes. Some commonly used formulations include Ham’s nutrient mixture F12, minimal essential medium, and Dulbecco’s modified Eagle’s medium (DMEM; Sigma-Aldrich, St. Louis, MO). Basic constituents of culture media include organic salts that maintain osmolality and act as pH buffers, essential amino acids needed for protein synthesis, vitamins for metabolic activities, and glucose for energy. To provide other factors needed for cell maintenance, serum is often added to culture media. Serum provides a broad spectrum of growth factors and other proteins including albumin. In lieu of serum, individual growth factors and proteins can be added to allow delineation of their individual effects in a given experiment [15].
15.2.4 Mechanics and Mechanobiology
It has been known at least since the time of Borelli (1608–1678) that mechanics plays important roles in biology. Nevertheless, it was not until the mid-1970s that it was shown experimentally that cells respond directly to changes in their mechanical environment, often via changes in gene expression. Indeed, more recently, it was even shown that matrix stiffness can contribute to stem cell differentiation. Whereas continuum biomechanics focuses on mechanical responses of cells, tissues, and organs to applied loads (under conditions of interest), mechanobiology focuses on biological responses of cells to mechanical stimuli. Mechanics and mechanobiology are thus allied fields—biological responses by cells can change tissue geometry, properties, and even loads, which in turn can alter the mechanical properties and hence, mechanical responses by that tissue, which in turn can change the mechanical stimulus sensed by the cell. Mechanobiology often involves transduction (i.e., conversion of a mechanical stimulus to a chemical signal), transcription (i.e., an associated change in gene expression), and translation (i.e., the resulting production of a protein). Clearly, therefore, systems biology is also a natural ally of biomechanics and mechanobiology, and there is a pressing need for multiscale models that address the multiple levels of response [16]. One of the key questions in mechanobiology is actually how the mechanical stimuli are transduced via corresponding signaling pathways [17]. Toward this end, there is a need for continued quantification of both the mechanical loads that act on cells and the subsequent biological responses, and to correlate how the former affects the latter. Results from studies on tissue equivalents promise to improve tissue engineering and regenerative medicine through proper mechanical and biological characterization [18].
15.3 Prior Experiments
15.3.1 Free-Floating Cell-Populated Lattice
Although observing cells plated on different ECM proteins can lead to the collection of considerable information, adherent cells behave differently within 3-D matrices than when attached to 2-D surfaces [19–22]. More than 30 years ago, Bell et al. introduced the circular, free-floating fibroblast-populated collagen lattice (FF-FPCL) [23]. Collagen gels containing embedded fibroblasts were suspended in culture media, and over a period of days, the cells compacted the lattices and thus reduced the gel diameter. The resulting tissue was noted as resembling the skin and having a rubber-like consistency. The rate and extent of compaction could be varied depending on a number of factors, including cell concentration, collagen density, cell passage number, and the presence of inhibitors of cell contractility such as cytochalasin B. Many investigators point to this work as the beginning of tissue engineering, but it also provided a simple experimental framework for studying cell–matrix interactions in a 3-D matrix.
The FPCL has been used predominantly as an in vitro model in wound healing research to examine cell–matrix interactions with an emphasis on understanding factors associated with wound contraction and closure. Detailed methods for the casting and culturing of FPCLs are described by Ehrlich [24]. Briefly, a solution containing known densities of collagen (usually type I) and fibroblasts is cast into a circular mold, often a bacteriological or tissue culture dish, allowed to undergo gelation, and then suspended in culture media. Over time (on the order of days to weeks), the embedded cells compact the collagen, which expels fluid from the matrix and reduces the overall volume of the lattice. The standard protocol described [24] results in an initial density of collagen ∼1.25 mg/mL and an initial concentration of fibroblasts of 50,000 cells/mL. The compaction of this standard configuration is characterized as occurring in two phases: an initial lag phase and a subsequent log phase (Figure 15.2) [25]. During the lag phase, there is little to no lattice compaction. This observation is likely due to the time required for the cells to begin adhering to and spreading within their surrounding matrix. After approximately 6–8 hours, lattices enter the log phase when the rate of compaction can increase greatly, then decrease gradually over multiple days until yielding an apparent steady state where compaction becomes minimal or ceases altogether. The compaction in this phase is believed to arise from cellular contractions (cycling of protrusion and retraction) associated with locomotion plus associated matrix reorganization. The degree of compaction is usually reported as a percent decrease in the circular surface area or diameter, which in standard experiments is on the order of 50–70%. These studies can be modified to examine changes in the rate of compaction, cell morphology and phenotype, and lattice organization due to a number of factors, including different cell types and concentrations, matrix compositions and densities, and the presence of exogenous growth factors.

In the initial experiments performed in Reference 23, differences in the rate and extent of compaction were noted when modifying either the collagen density or number of fibroblasts. Increasing the collagen density while using the same number of cells resulted in slower compaction, with the final extent of compaction reduced. The cessation of compaction is considered by some as a state of equilibrium at which the size and organization of collagen fibrils or fibers prevent further compaction [24]. For lattices with higher collagen densities, cells are surrounded locally by more collagen and can achieve such an equilibrium without reducing the overall volume of the lattice to the same extent as a lower density matrix.
Conversely, if the collagen density is held fixed and fibroblast concentration is increased, both the rate and the extent of lattice compaction increase [23]. When the cell concentration is increased in the standard protocol by an order of magnitude (0.5 × 105 to 5.0 × 105), the lag phase is decreased by 4–6 hours, and the log phase is completed after 6 hours rather than the 24 hours needed for the lower densities [25]. This change in the compaction profile is thought to be caused by forces generated by recently passaged cells that are spreading and elongating. Though spreading-related forces are weak when compared with cellular forces linked with migration and especially contractility, increased cell densities can increase greatly the total force applied to the lattice during cell spreading [26]. Of course, these forces may only affect the matrix in the immediate vicinity of a spreading cell. Yet, at high cell concentrations, individual cells may reside within close proximity to each other such that many collectively applied forces are exerted on the same volume of matrix during initial spreading.
Different cell types produce different compaction rates and final degrees of overall compaction. Fibroblasts from different species as well as those from different tissue sources compact collagen at varying rates [27]. Increased passage number for a given cell line has also been shown to reduce the rates at which collagen is compacted [23,27]. For example, when comparing normal rat skin fibroblasts with a line of transformed rat sarcoma cells, it was found that normal cells compacted the matrix much more than the transformed line did [28]. By 7 days, however, the total extent of compaction was approximately equal for both cell lines and remained equal for the remaining period of culture (14 days). This pattern of compaction is likely due to differences in cell proliferation, as the number of transformed cells at 7 days was almost 100 times greater than the normal cells. When comparing bovine vascular smooth muscle cells with human dermal fibroblasts, the latter compacted gels more quickly and to a greater extent [29]. This difference is possibly due to the fibroblasts having a more elongated morphology compared with the smooth muscle cells, which would allow cellular locomotion and contractility to influence more surrounding matrix. Aortic adventitial fibroblasts and medial smooth muscle cells from the cynomolgus monkey were found to compact collagen lattices similarly over a 24-hour period [30]. Cells from different aged donors as well as those from pathological conditions can also exhibit differing degrees and rates of compaction [31]. Smooth muscle cells isolated from balloon-induced intimal thickening in rat aortas compacted collagen to a lesser degree than smooth muscle cells from the underlying media or from normal aortas. If cultured in plasma-derived serum instead of fetal bovine serum, compaction was decreased for all cell types; plasma-derived serum similarly resulted in less compaction by newborn (4-day-old) rat smooth muscle cells compared with those from young (8–10 weeks) and old (16–18 months) adult rats.
Matrix composition similarly plays an important role in the rate and extent of the cell-driven compaction of lattices. Multiple fibroblast lines have been shown to compact lattices comprised of different collagen types at different rates and to varying degrees [32,33]. Dermal fibroblasts compact type III collagen more quickly and to a greater extent than type I, while type II collagen is compacted more slowly and to a lesser degree than either type I or type III [32]. These cells had similar morphologies across all three collagen types, however. Yet others have found the opposite to be true with MRC5 fibroblasts, compacting type I collagen more than type III [33]. These differences could be attributed to a number of factors, including duration of pepsin digestion during collagen isolation, differences in initial collagen and cell densities, and potential differences between dermal and MRC5 fibroblasts [32–34].
Dermal fibroblasts also tend to compact collagen lattices to a greater extent than fibrin lattices, whereas gingival fibroblasts compact collagen to the same degree as dermal fibroblasts while compacting fibrin more and completely degrading it after 7 days in culture [35]. The addition of ε-amino-caproic acid (an inhibitor of fibrinolysis) to gingival fibroblast–fibrin cultures resulted in compactions similar to the untreated dermal fibroblast–fibrin lattices. This finding suggests that gingival fibroblasts may have increased fibrinolytic capability compared with dermal fibroblasts.
The addition of GAGs and PGs to collagen lattices can affect the compaction of the lattices. The addition of hyaluronan to collagen lattices has been shown to increase the degree to which smooth muscle cells can compact the matrix compared with collagen alone [30]. When CD44 binding of hyaluronan is blocked, compaction is comparable to collagen alone, which indicates that the cells may be able to use hyaluronan as an indirect linker to collagen to expedite matrix compaction. In contrast, the addition of decorin can reduce lattice compaction by hypertrophic scar fibroblasts, likely through the sequestration of TGF-β1, which is highly expressed by these cells [36].
The ability of cells to cross-link collagen is also important in the compaction of collagen lattices. Collagen is enzymatically cross-linked primarily through the action of lysyl oxidase, but can also be cross-linked by transglutaminases [37]. Pretreatment of dermal fibroblasts with a lysyl oxidase inhibitor, β-aminopropionitrile (BAPN) delayed the onset of compaction, and subsequent exposure during culture reduced the final degree of compaction [38]. If BAPN is added only during lattice compaction, lung fibroblasts maintain normal levels of compaction early in culture (∼2 days) but exhibit reduced compaction at later times compared with untreated lattices [39]. Early compaction of the lattices may proceed normally assuming that BAPN does not inhibit cell spreading or attachment to surrounding collagen. That is, the cells may continue to actively pull in collagen and compact the matrix. With the inhibition of lysyl oxidase, however, prior data suggest the cells are unable to covalently cross-link the collagen following the initial compaction, and thereby, they cannot entrench any of applied deformations within the matrix. In the normal culture environment, cells could cross-link their surrounding collagen to entrench the local compaction, then detach and adhere to other collagen to continue the process of compaction and cross-linking. Cross-linking may thus allow resident cells to develop a residual matrix tension in an attempt to stiffen their local matrix environment to achieve a preferred mechanical environment; this process or mechanism has been referred to as tensional homeostasis, which appears to be an in vitro example of a general process of mechanical homeostasis that promotes tissue formation, maintenance, remodeling, and adaptation [40–42].
Compaction of collagen lattices by fibroblasts also depends on specific integrin–matrix interactions [43]. Multiple reports have shown that fibroblast compaction of lattices formed from reconstituted type I collagen is achieved primarily through cell–matrix interactions via the α2β1 integrin [44–46]. Altered matrix composition can allow compaction even in the presence of α2β1-directed antibodies through cell attachment to other matrix components that are entangled with the collagen [30]. Moreover, cells that are deficient of the α2β1 integrin are still capable of compacting collagen lattices via the αvβ3 integrin [47,48]. This difference in adhesion can also lead to changes in mechanobiological and biochemical responses as, for example, PDGF increases compaction in α2β1-mediated gels but not in those mediated via αvβ3 [47].
The culture environment of collagen lattices can greatly affect their compaction. It has been shown that serum is required for the compaction of FPCLs, likely due to the presence of myriad growth factors [28,49]. Lattices cultured in the absence of serum can also compact if the culture media is supplemented with growth factors such as TGF-β1 and PDGF [50–53]. Compaction of lattices exposed to PDGF suggests that part of the process may be mediated through cell migratory processes [52,54]. Exposure to TGF-β1 leads to lattice compaction, but the stimulated cellular mechanism may be augmented when cells express the αPDGF receptor [50,53]. This suggests that TGF-β1 may induce PDGF release and subsequently increase compaction [53,55]. TGF-β1 has also been shown to increase the expression of integrins, which would allow cells to adhere to more collagen and to transmit more force to the matrix [56,57]. TGF-β1 is also necessary for fibroblasts to differentiate into the more contractile myofibroblasts, thus increasing the contractile force they can apply to their surrounding matrix in the free-floating collagen lattice, possibly through increased actin expression [11,58].
Gene and protein expression have been examined in free-floating lattices and are usually compared with levels expressed by cells in lattices that are allowed to adhere to the surface of the culture dish. It has been assumed by many that adhered matrices develop a tensile mechanical environment while free-floating gels remain mechanically relaxed, though the mechanical environment has not been explicitly measured or derived [11]. Cells in floating lattices have a reduced response to PDGF compared with adhered gels as measured by level of receptor autophosphorylation [59]. Type I collagen expression is also decreased in floating constructs compared with monolayer cell cultures while MMP-1 levels are increased [45]. Blocking α1β1 and α2β1 integrins further downregulates type I collagen expression and upregulates MMP-1 in floating gels [45]. Expression of MMPs by ocular fibroblasts has similarly been shown to change throughout culture time [60]. MMP-1 expression is elevated at 9 hours and increases to day 1 before dropping off by day 7. MMP-2 and MMP-3 showed similar trends; however, total expression of both at 9 hours is markedly lower compared with MMP-1, with MMP-2 levels being significantly higher than MMP-1 and MMP-3 at day 7. Total protein measures for MMP-2 were also much greater than levels of MMP-1 and MMP-3. The varying levels of MMP activity throughout lattice compaction could possibly arise from a continually evolving mechanical environment. In examining cellular contractile components, anchored and floating lattices show similar levels of β-actin expression, but anchored gels show higher levels of α-SMA. In both configurations, TGF-β1 increases the ratio of α-SMA to β-actin [51].
Measures of gene and protein expression show that there are global differences between adhered and floating lattice cultures, but such measurements do not account for the potential of local variations within the matrix. Free-floating lattice compaction has been shown to lead to regional variations in cell and matrix alignment as well as cell phenotype [25,61]. After 24 hours in culture, two distinct fibroblast phenotypes and states of matrix organization can arise. The center of the lattice is populated by randomly oriented fibroblasts with densely compacted, randomly oriented collagen, whereas the periphery of the gel contains fibroblasts that have differentiated into myofibroblasts with both cells and collagen aligned parallel to the outer edge of the lattice. Similar results were shown by Costa et al. [62] in various constrained geometries—cell compaction of collagen lattices leads to fiber alignment parallel to any free or unconstrained edge (Figure 15.3). Although there are no externally applied loads or constraints on the system, the peripheral alignment is potentially due to the development of different local mechanical environments that could lead to differences in local gene and protein expression.

15.3.2 Uniaxial Collagen Gels
Externally applied mechanical loads can greatly alter cellular activity, and tissue equivalents represent controllable systems suitable for examining the mechanobiological responses of cells to such applied loads. Uniaxially constrained collagen gels are simple systems wherein the axial, or in-line, force can be measured and the axial strain can be controlled accurately and precisely. One of the first experiments carried out on uniaxial tissue equivalents sought to characterize cellular forces associated with locomotion [63]. A collagen solution containing either normal or diseased skin fibroblasts from human or calf explants was cast in a rectangular mold and allowed to polymerize around Velcro constraints; one end was connected to a strain gauge to infer the force generation and the other to a mobile vernier to adjust the length of the gel. It was reported that tension developed within 30–60 minutes of polymerization and increased rapidly over 6–12 hours with increases in tension slowing or ceasing by 24–48 hours. During the period of observation, the geometry of the gel changed due to cellular compaction, including development of a parabolic shape (maximal gel width at the end restraints, minimal width at midaxial location) and reduction in thickness. After tension reached a steady state, the gels were lengthened or shortened to cause a step increase or decrease of 2 g of load. The cells restored the in-line force to the previous steady level in both cases within 1 hour.
This investigational setup has been modified, characterized, and used by multiple groups [41,64,65]. It can be used to perform experiments similar to those for the free-floating lattice to examine the effect of different initial or continual culture conditions on the mechanobiological responses to uniaxial constraints. Easy to implement variations include different cell types, cell concentrations, matrix type, matrix composition and density, static or cyclic loading conditions, and the effects of different growth factors and pharmacological agents. For example, Delvoye et al. noted that calf skin fibroblasts generated larger forces compared with human skin fibroblasts [63]. They also observed that calf dermatosparactic skin fibroblasts were less effective at generating tension within the uniaxial lattice when compared with normal fibroblasts, which likely results from reduced cell–matrix interactions observed previously in animals [66].
A similar experimental setup has been employed to measure forces generated by endothelial cells [64]. Instead of casting the cells within the collagen, an acellular collagen solution was cast, and following gelation, endothelial cells were seeded on the apical surface to form a monolayer. Whereas fibroblasts were found to reach a steady state force within 2 days, endothelial cells required 4–5 days in culture and only reached force levels an order of magnitude lower than the fibroblasts. This finding could have been due to the different casting methods, though no corresponding experiments were performed with fibroblast monolayers. Uniaxial equivalents also allow direct comparisons between cell types. For example, they have been employed to compare and characterize differences between human dermal and Tenon’s capsule (ocular) fibroblasts [67]. The ocular fibroblasts showed a gradual increase in force generation that achieved only one-third of the force generated by dermal fibroblasts after 24 hours.
Tissue origin can also play a role in cellular responses as smooth muscle cells from different layers of piglet pulmonary arteries developed tensile forces in uniaxial tissue equivalents at different rates and to different extents in 24 hours [68]. Cells from the outer medial layers generated force more quickly than those from inner layers and they also generated more force. Cells sourced from hypoxic animals generated smaller forces overall, with cells from the inner media producing more tension than those from the outer layers.
Free-floating lattices have increased rates of compaction when cell concentrations are increased; the same holds for uniaxial tissue equivalents. Along the same line, increased collagen density increases the rate of force generation in tethered gels while reducing the rate and extent of compaction in free-floating constructs [63]. Increasing collagen density would increase initial substrate stiffness and apparently require less cellular compaction to achieve homeostatic or preferred forces [41]. The role of initial cell and matrix densities is likely important when trying to engineer potential regenerative therapies as well [69]. Too high of a cell seeding can lead to overly compacted and damaged constructs.
Uniaxial tissue equivalents also allow measurement of contractility by cells [64]. Similar to Reference 63, force generation began within 3 hours of casting fibroblast populated uniaxial tissue equivalents, increased rapidly over 24 hours, and reached a steady level in 48–72 hours that was maintained in cultures taken out to 7 days. To assess contractility, constructs were exposed to thrombin after reaching steady state, which induced an increase in force within 5–10 minutes that was sustained for several days. Once exposed to cytochalasin D to disrupt actin filaments, all tension in the system was lost within 10 minutes.
Whereas it was expected that disruption of actin would diminish tension development, it appears that microtubules can also influence cellular forces applied to matrices [64,70]. Disrupting microtubules within fibroblasts leads to an initial increase in force in uniaxial constructs that is gradually returned to prior steady-state levels. Microtubules help maintain cell shape and buffer the tension of actin filaments by acting as compressive supports. When disrupted, the tension in the actin cytoskeleton can no longer compress the microtubules, hence, leading to a net increase in the tensile force on the tissue equivalent. Over time, the cells seem to want to maintain a homeostatic level of tension and potentially relax intracellular tension to restore preferred levels [41,70].
As stated previously, cells appear to establish and then maintain a preferred level of tension, which is referred to as tensional homeostasis [41]. By cycling the length of uniaxial tissue equivalents, it became clear that the cells sought to restore at a preferred force level. On reaching steady state, constructs were then shortened abruptly, which resulted in a sharp decrease in force. Almost immediately thereafter, however, the force began to increase quickly before plateauing at a steady-state value. Lattices were also cycled between lengthening and shortening. The cycling regimen started with an applied 0.6-mN increase above endogenous force, then followed a pattern of a 15-minute rest, a 1.2 mN unloading over 15 minutes, 15-minute rest, and a 1.2 mN loading over 15 minutes. This protocol kept the cycled force measurements centered about the endogenous force. Other variations of this cycling regimen have been used to cycle from the endogenous tension level to either 1.2 mN above or below it. When constructs were lengthened, force increased throughout loading and then immediately started to decrease during the resting cycle; when they were shortened, forces decreased and then gradually started to increase once at rest. These results again suggest that cells attempt to maintain a certain mechanical environment and they respond quickly to perturbations to actively restore a preferred state.
The idea of tensional homeostasis is supported further by the work of Marenzana et al. [40]. After adding cytochalasin D to release fibroblast-induced forces at various time points in culture, it was found that not all tension is released from the matrix and the amount of this residual matrix tension seems to increase linearly between 4 and 60 hours in culture. During this time, the total axial force in the uniaxial tissue equivalents reaches a steady level in 24 hours. It appears that the cells actively deform the matrix in an attempt to restore tensional homeostasis. To aid in this process, they will also remodel their resident matrix to build in tension (the aforementioned residual matrix tension) and potentially reduce the amount of active force they need to apply to maintain the preferred mechanical environment.
Growth factors and cytokines can also alter cell contractility and matrix compaction in uniaxial constructs. In particular, TGF-β1 has been investigated extensively [40,71,72]. TGF-β1 tends to increase the rate and extent of force generation, although it has been shown that high concentrations of this cytokine could potentially inhibit increases in force [71]. Exposure to TGF-β1 also appears to speed the development of the residual matrix tension and to increase fibroblast contractile forces greatly, possibly by inducing the myofibroblast phenotype [40]. TGF-β1 also alters cell responses to lengthening of the tissue equivalent as treated fibroblasts initially increase then reduce the tension relative to controls, which begin reducing matrix tension almost immediately following the perturbation [72].
The uniaxial mechanical environment can also lead to the up- or downregulation of multiple genes and proteins. If constructs are prestrained to increase matrix stiffness before the onset of force generation, the expression of multiple genes can be altered [73]. Prestrain significantly increases the expression of MMP-2 and tissue inhibitor of metalloproteinase (TIMP)-2 in human dermal fibroblasts while collagen type III expression is increased only at a high prestrain (10%), though not significantly due to a broad range of expression in control expression. Cyclic loading (one cycle per hour) of dermal fibroblast-populated constructs also leads to changes in protease expression [74,75]. MMP-2, MMP-9, and tissue plasminogen activator (tPA) levels are increased by cyclic loading while MMP-3 and urokinase-type plasminogen activator (uPA) levels are reduced. These levels can also be altered by construct geometry [75]. The upregulation of both MMPs and TIMPs due to increased mechanical loads suggests that the resident cells attempt to remodel the constructs to reduce the amount of tension in the system and restore tensional homeostasis.
15.3.3 Biaxial Collagen Gels
While an extensive number of studies have been carried out employing free-floating and uniaxially constrained collagen lattices, biaxial tissue equivalent-based investigations have been relatively few in number. Most of this work has focused on the design and characterization of biaxial systems, measurement of cell and mechanically induced tissue anisotropy, and mechanical characterization of equivalents following prescribed culture periods [76–78].
Similar to uniaxial tissue equivalents, a collagen solution containing cells is cast in a mold containing restraints, usually a porous plastic, to facilitate mechanical manipulation of the constructs. Two mold shapes are usually employed, either a square with restraints on each side or a cruciform shape with restraints at the end of each arm [76,77]. On gelation, the specimens can be mechanically loaded in culture either through the attachment of weights to the restraining bars to prescribe an isotonic load or by holding the specimen dimensions static and allowing the resident cells to compact the matrix isometrically and thereby develop endogenous loads [76,77]. Biaxial systems also allow multiple loading protocols, including equibiaxial stretching or loading (i.e., same stretch or force along each axis), non-equibiaxial stretching or loading (often proportionally), or strip biaxial stretching (one axis held fixed at original length while other axis is loaded).
Measuring biaxial strains in the central region (or arms of the cruciform specimens) can be achieved by tracking the positions of embedded markers within the gel, often microspheres [76]. The tracking of marker positions during both cell-mediated compaction and mechanical testing allows calculation of the deformation gradient tensor, F, in 2-D and calculation of associated strain measures. Other groups have also used histological stains or ink on the specimen surface to track strains [78]. It has been shown that strain is often inhomogeneous across biaxial specimens during cell compactions, though they may be nearly homogeneous within the central region of the specimens [76].
Boundary conditions in culture can be used to change the organization of collagen fibers. In comparing the structure and mechanical behavior of biaxially and uniaxially constrained gels, it has been shown that uniaxial constraints result in a marked increase in tissue anisotropy following 3 days of cell-driven remodeling [79]. In biaxial gels, collagen fiber orientations remain randomly oriented in the central region during equibiaxial stretching or mechanical testing, indicative of an isotropic response. In contrast, uniaxial gels develop a preferred collagen alignment parallel to the constrained axis, which thus exhibits a markedly stiffer response in mechanical testing compared with the unconstrained axis.
The degree of tissue anisotropy may also be altered by imposing unequal initial stretches to constructs [77]. Applying two different magnitudes of stretch to each axis will increase collagen alignment toward the direction of larger initial stretch. With a cruciform-shaped sample, it is noted that the mechanical environment of the arms is uniaxial and thus, results in distinct alignment parallel to the corresponding axis regardless of the biaxial loading protocol. Moreover, if the arms of one axis of a cruciform sample are wider than the other axis, the fibers tend to align in the center of the tissue equivalent toward the axis of the wider arms [78]. As the ratio of widths of the arms is increased, the degree of alignment also increases. This finding is most likely due to a greater force generated by the larger number of cells in the wider arms of the gel compared with the narrower arms.
The application of external loads also leads to some initial alignment of collagen fibers [77,80]. As stated before, uniaxial constraining leads to alignment along the constrained axis during cell-mediated compaction. If that axis is then unloaded and the gel constrained or loaded perpendicular to the original constraint, the collagen lattice can become isotropically distributed [80]. If cultured out further, the cells will begin to reorganize the matrix and align collagen predominately with the new constrained axis. Polarized light microscopy has also been employed to monitor fiber alignment even during biaxial mechanical testing [81–83]. Displacement along one axis shows a shift in collagen fiber alignment toward the direction of the applied motion [81].
Fibroblast-populated fibrin tissue equivalents have also been used to examine the role of fiber alignment in a biaxial constrained environment [84]. After 10 weeks in culture, fibrin-based tissue equivalents showed marked changes in tissue composition. Collagen accounted for approximately 3–4% of the total dry weight of cruciforms at 10 weeks, with low levels of elastin present (∼0.10–0.15% dry weight). Regional differences were also found, with the most pronounced being a higher percentage of collagen content in the narrow arms of cruciforms with geometrically induced fiber alignment.
15.4 Prior Mechanical Analyses
Given that embedded cells are highly responsive to their mechanical environment and changes therein, there is clear motivation to quantify the states of stress and strain imposed on a tissue equivalent during culture, as well as to quantify changes that result due to the action of the cells on the matrix. It is surprising, therefore, that appropriate mechanical quantification remains wanting in many regards. Indeed, not only has there been little attention to the macroscopic stress or strain fields, there has been less attention to the cell–matrix interactions.
15.4.1 Free-Floating Lattices
The first mechanical model of the free-floating collagen lattice sought to quantify the traction force associated with the cell [85]. A spherical geometry was employed as spherical symmetry simplifies both the problem formulation and solution. The ECM was modeled as a linear viscoelastic material described by the following stress–strain–strain rate relation:
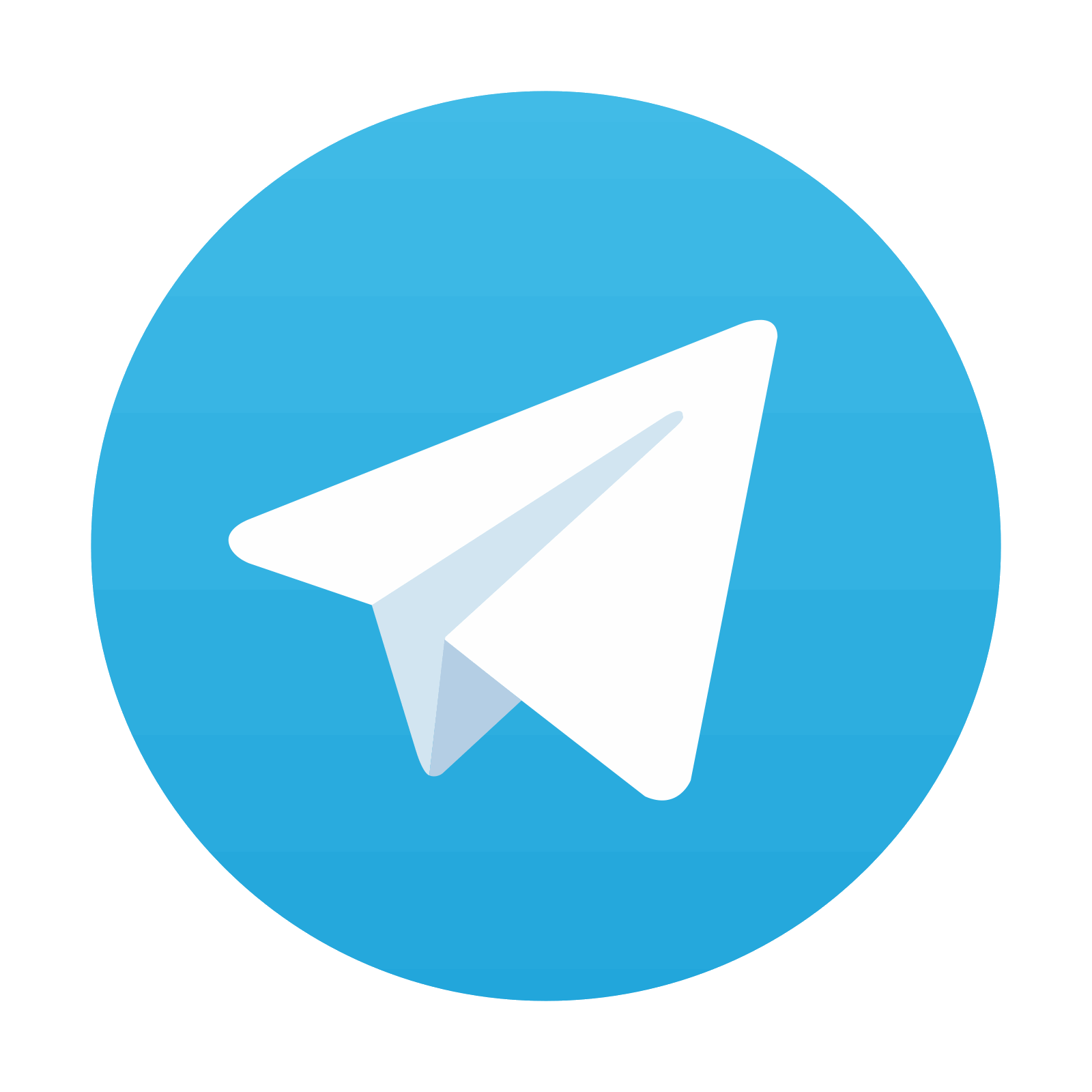
Stay updated, free articles. Join our Telegram channel
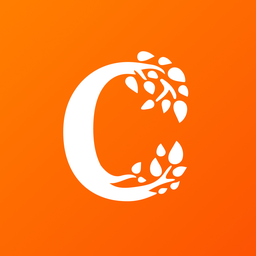
Full access? Get Clinical Tree
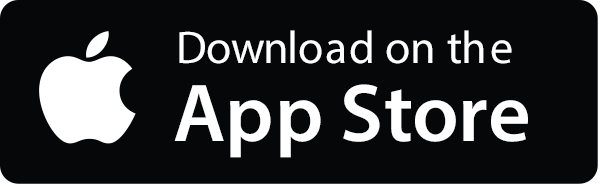
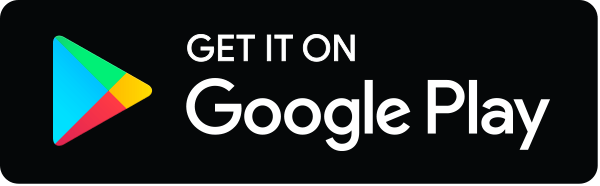