Strategies and Challenges for Bio-inspired Cardiovascular Biomaterials
Department of Biomedical Engineering, Boston University, Boston, MA, USA
Department of Biomedical Engineering, Boston University, Boston, MA, USA; Department of Materials Science and Engineering, Boston University, Boston, MA, USA
13.1 Need for Cardiovascular Biomaterials
13.1.1 Cardiovascular Tissue Regeneration
Harnessing the cell’s capacity to renew, revive, or restore is a hallmark of regenerative medicine. Unfortunately, the human heart and blood vessels have little true regenerative capacity, especially when injured. Despite many improvements to biomaterials and major advances in stem cell biology, nature’s sophisticated hierarchical organization and adaptability is still the benchmark achievement that modern biomedical engineering technologies seek to replicate. Bio-inspired cardiovascular tissue engineering strives to apply structure–function relationships in the development of biological substitutes to restore, maintain, or improve tissue function [1]. We review here the current techniques for bio-inspired cardiovascular materials and the milestones we as a field have yet to reach.
13.1.2 Unmet Clinical Need: Incidence and Prevalence of Cardiovascular Disease
In its 2013 update, the American Heart Association estimated that 83.6 million American adults (more than one in three) have one or more types of cardiovascular disease (CVD), which encompasses high blood pressure and coronary heart disease (myocardial infarction [MI], angina pectoris, heart failure, stroke, and congenital heart defects) [2]. A global health problem, CVD impacts all races, genders, ages, and socioeconomic groups, with an average of one death every 40 seconds in the United States alone. Atherosclerosis—the underlying systemic disease in which inflammation results in fatty plaques and scar tissue build up along artery walls—leads to many coronary events (e.g., MI, stroke); an estimated 397,000 coronary artery bypass graft (CABG) procedures were performed in 2010 alone.
In addition to CVD that can be managed with preventive measures, between 4 and 10 per 1000 live births in the United States will also present with congenital heart defects (i.e., structural problems resulting from abnormal formation of the heart or major blood vessels) [3,4]. Although some minor defects may resolve spontaneously, an estimated 800,000 American adults were thought to be living with a congenital heart defect in 2000, some whose disease went undetected during childhood [5]. Pediatric patients with major malformations are usually treated surgically, but tissue availability is limited and the current clinical options do not grow with the child. Health expenditures for CVD were estimated at $312.6 billion for 2009 [2]; by comparison, the estimated cost of all cancer and benign neoplasms was $228 billion in 2008.
13.1.3 Need for Tissue-Engineered Solutions
MI can lead to myocardial necrosis or loss of viable myocardium that is thinned or fails to contract [6]. The infarction can take weeks to months to heal, but loss of terminally differentiated cardiomyocytes (often 1 billion or more) [7,8], the remodeling process, and resulting scar tissue may limit function [7]. Moreover, the environment of the stressed heart may lead to maladaptive remodeling that can lead to arrhythmogenesis, heart dilatation, wall thinning, and decreased cardiac output [9–12]. Although heart transplantation remains the most viable option for end-stage heart failure patients, donor hearts remain limited (3.6 per 1000 deaths in 2010) [13]. Ventricular assist devices and other mechanical circulatory support have a projected duration of 5 years, but still have associated thrombogenic issues. Although allografts avoid thromboembolisms, immunogenicity has led to degenerative changes (e.g., calcification) and eventual graft loss within 8–10 years [14]. Because autologous cell sources avoid rejection issues and the need for immunosuppressive medication [15,16], autologous myoblasts and other multipotent adult stem cells have been implanted clinically, but arrhythmias and exacerbating inflammation remain long-term concerns [17]. Although direct cell injection of embryonic stem cells (ESCs) has shown some improvement to cardiac function, approximately 90% of cells are lost in circulation [18], and impure cell populations still carry risk of teratoma formation [8,19]. Thus, tissue-engineered myocardial patches aim for (a) targeted delivery of pure cell populations, (b) integration with three-dimensional (3-D) structure and mechanical compliance with fatigue resistance, (c) synchronous electrical integration of viable cells, and (d) rapid vascularization to support cell survival.
For vascular grafts, autologous vessels are most desirable as blood vessel substitutes in coronary bypass procedures and other small-caliber blood vessel replacements (<6 mm). However, because of trauma, disease, or prior surgeries, suitable autologous vessels (e.g., saphenous vein or internal mammary artery) are unavailable in >10% of patients [20,21]. Success with cryopreserved cadaveric grafts, umbilical vein grafts, and arterial allografts has also been limited [22–26]. Although effective for large vessel replacements with high flow and low resistance, synthetic materials (e.g., Dacron, expanded polytetrafluoroethylene [ePTFE]) display suboptimal behavior and have high risk of thrombosis in smaller vessel replacements [27–29]. Additionally, vascular grafts have had problems with intimal hyperplasia, accelerated atherosclerosis, and compliance and diameter mismatches at the anastomoses [30–34]. Ideal tissue-engineered vascular graft substitutes require (a) proper mechanical properties, such as burst pressure and viscoelastic properties [35]; (b) contractile response or vascoactivity [36,37]; (c) nonthrombogenicity [37]; and (d) responsiveness to postimplantation remodeling by the host tissue [38].
In addition to the clinical need for cardiovascular replacements, tissue engineering offers strategic advantages as model systems to investigate specific biologic questions [39]. The ability to manipulate composition, geometry, and microstructure in a controlled environment that can be monitored makes tissue-engineered structures (e.g., organ-on-a-chip) more ideal than biologic explants in the study of cell–matrix interactions, cell and matrix mechanics, and mechanobiology. Furthermore, in combination with the technological advances in computational modeling, more complex questions can be addressed in understanding the pathology of CVD or in tissue model development for drug screening, to which we refer to other more in-depth reviews [40–44]. Computational modeling that predicts and validates experimental results could shed new light on structure–property–performance relationships. Likewise, we refer to other reviews for in vivo experimental models that can be used to assess tissue-engineered cardiovascular constructs [45–49].
13.2 Structure Equals Function: Focus on Strategies that Introduce Hierarchical Organization
13.2.1 Tissue Engineering Tools
Although the immediate clinical goal is to restore functionality (e.g., porcine valve replacements in pediatric patients despite lack of native leaflet trilayered structure), the long-term goal of tissue engineering is to manipulate cells and biomaterials to develop constructs that recapitulate native tissue composition and structure to guide postimplantation remodeling, growth, and self-maintenance. Generally, taking a bottom-up assembly approach and building materials up molecule by molecule to form supramolecular architectures and integrating intrinsic (e.g., gene manipulation) and extrinsic (e.g., mechanical conditioning) cues, tissue engineering seeks to incorporate hierarchical organization into the tissue structure using (Figure 13.1):
- Scaffolds, which provide the foundational architecture and mechanical properties, and the inactive structure on which cells act
- Bioreactors and bioactive molecules, which provide short-term active exogenous forces (i.e., biochemical and biophysical cues) to which the cells must react
- Cell sources, of which the specific cell phenotype and its subsequent extracellular matrix (ECM) remodeling capabilities provide both short- and long-term regenerative agents.

Although at one point, material scientists sought to make inert biomaterials for implantation, as we continue to learn more about the biologic response to implants (e.g., inflammation, thrombus formation), the new paradigm in tissue engineering is to create more complex, active biomaterials that can interact with cells, bioactive molecules, and its microenvironment to initiate an appropriate host response [50]. Instead of avoiding inflammation, we may now engineer the physical characteristics of biomaterials to promote cell invasion and a modulated inflammatory or immune response to elicit desired remodeling (e.g., enzyme degradation, monocyte activation). We may also modify the surfaces with therapeutic agents (e.g., drug delivery, immobilized growth factor) to elicit a specific response.
In constructing structural tissues in vitro, tissue engineers must lay enough of a structural template for cells to remodel the injured structure at the target site before achieving compositional and functional integration with the surrounding environment [39,51,52]. The choice of scaffold must maintain its mechanical integrity as the cells entrapped within or invading the scaffold produce enough ECM to rebuild the compromised structure, a process that usually takes days. Once structural remodeling has taken place, compositional remodeling can occur, in which the scaffolding can be broken down and replaced simultaneously by cell-produced ECM, a process that can take weeks to months. Table 13.1 lists some examples of parameters to consider when developing tissue-engineered constructs.
Table 13.1 Some Design and Remodeling Parameters to Consider before and after Implantation [39,63,73,76]
What parameters can be controlled before implantation? | What parameters must be accounted for after implantation? | |
---|---|---|
Scaffold |
|
|
Bioreactors and bioactive molecules |
|
|
Cell sources |
|
|
13.2.2 Hierarchical Structure of the Heart and Blood Vessels
The basic hierarchical organization in the heart and blood vessels is seen in Figure 13.2. The heart is composed of striated muscle organized into anisotropic laminae that wrap around ventricular cavities [53,54]. Three major cell types comprise cardiac tissue: cardiomyocytes, fibroblasts, and endothelial cells (ECs) [55,56]. Cardiomyocytes form the contractile myocardium layer—the most difficult layer to replicate—at approximately 2 cm thick; high oxygen and nutrient demand is a factor in constructing viable tissue. Several nonmuscular structures are embedded within these layers: neural and vascular networks, collagen fibrils, and fibroblasts [53,57–60]. To maximize peak force generation, structural organization and cytoskeletal and sarcomere alignment are critically important [53,61]. Fibroblasts provide ECM proteins and paracrine factors [55], while ECs line vasculature to prevent thrombus formation [56].

Blood vessels have three layers—tunica intima, media (contractile), and adventitia—that vary in thickness according to the size and type of vessel [62,63]. In the tunica intima, ECs align and orient in the axial direction in the lumen, in contact with blood flow and attached to the basement membrane connective tissue, to regulate vasomotor tone and solute diffusion. The media layer is composed mostly of sheets or bundles of smooth muscle cells (SMCs, containing actin and myosin filaments) oriented in the circumferential direction to resist pulsatile flow. SMCs can operate under either a contractile or a synthetic (promotes ECM secretion) phenotype [64,65]. The media is reinforced by elastin embedded between small amounts of collagen fibers, oriented in the axial direction, with the ratio of elastin to collagen varying from 0.25 to 1.5, depending on the artery type [66]. The wavy, undulating structure of elastin straightens under loads at physiologic pressures; collagen fibers straighten in <10% of fibers, but become load bearing above normal physiologic pressures; and distension is limited by stiffness as collagen exhibits nonlinear viscoelastic behavior [67]. The adventitia is a collagenous ECM layer of fibroblasts and nerves that serves mostly to give rigidity to the vessel. Tissue engineering focuses on the media, as this layer contributes mechanical strength and elasticity to the vessel, followed by the vasoactive intima.
The most commonly found collagen in cardiovascular structures, type I collagen forms the biomechanical scaffold on which cells attach [62,68]. Collagen has a Young’s modulus that ranges 5–10 MPa and provides the strength to resist failure at high pressures [66]; elastin has a Young’s modulus of 0.4–1 MPa and provides reversible extensibility, with a stretch ratio of 1.6, and fibers can maintain elastic properties up to 140% of extension [69]. However, despite its assumed necessity for viscoelasticity, elastin is often absent in engineered grafts because elastin is minimally expressed in adults [70] and because the purification process is labor-intensive [71]. Additionally, because tropoelastin (i.e., soluble monomeric form of elastin) must aggregate on the cell surface and be cross-linked via helper proteins, recreating the complex temporal, spatial, and structural interactions to form functional elastic fibers remains a significant challenge [67,72].
13.3 Tissue Engineering Approaches to Cardiovascular Biomaterials
13.3.1 Scaffolds: Foundational Architecture and Mechanical Properties
Scaffolds provide the initial 3-D pore structure for cells to adhere, migrate, proliferate, differentiate, secrete ECM, and apoptose [73]. Over the long term, scaffolds can also release biochemical signals to maintain cell phenotype, induce differentiation, and influence metabolism. The scaffolding composition and its subsequent cellular remodeling can affect the cell shape, cytoskeleton, elastic modulus [74], and mechanotransduction of cells [75]. Thus, the design of the scaffolding material itself plays a significant role in controlling a tissue-engineered construct’s architecture and mechanical properties as cells act upon scaffolding structures to self-organize its ECM.
Current tissue-engineered cardiovascular constructs often integrate unsuccessfully because grafts breakdown from either (a) failure from the repetitiveness or magnitude of the physiologic loads or (b) lack of oxygen and nutrient transport to implanted cells [76]. Transport by diffusion can only support four to seven cell layers (approximately 100–200 μm thick) [77–81]. Efforts of using scaffolds that recreate native cardiovascular tissue architecture and mechanical properties fall into one of the following categories: synthetic polymers, natural ECM, and decellularized matrix. Examples of each biomaterial and its corresponding advantages and disadvantages are listed in Table 13.2.
Table 13.2 Overview of Biomaterials Used for Scaffolding Structures for Cardiovascular Tissue Engineering (Adapted from References 63, 76, 93, and 200)

Isotropic porous scaffolds have inadequate mechanical strength for load-bearing tissues like the heart and blood vessels. Structural anisotropy is necessary for directing blood flow, as well as for excitation propagation in cardiac muscle; however, engineering constructs with aligned cells has been challenging. The methods in which biomaterials are fabricated or processed can influence the resulting anisotropy (e.g., forming fibers or sheets), as well as cell adhesion (e.g., passivating biomaterials or changing hydrophobicity) and other mechanical properties [82]. We focus our discussion on methods of introducing anisotropy via topographical cues using contact guidance principles using (a) soft lithography on the nano- and microscale, (b) electrospinning to encourage self-assembling tissues, and (c) decellularization to preserve existing ECM topological and molecular cues of tissues.
To introduce hierarchical structure to tissue-engineered constructs, the topography of the actual scaffold provides physical contact guidance cues to recapitulate the spatial cues of the microstructure in the native environment. Tissue engineers often use soft lithography techniques to easily create and inexpensively reproduce various elastomeric stamps, molds, and masks (see reviews elsewhere) to introduce physical topographies into constructs (Figure 13.3) [83–85]. Using soft lithography to mold topographical substrates (usually polydimethylsiloxane [PDMS]) that mimic physical native features, contact guidance has been shown to be an important factor in inducing vascularization in biomaterials, even without using growth factors [39]. Host cells have been able to penetrate membranes with pore sizes ranging from 0.8 to 8 μm [86], while studies found the optimum pore size was approximately 60 μm [87,88]. Elastomeric scaffolds have been able to recreate the aligned cardiac cell structures and physiological mechanical properties using accordion-like honeycomb-shaped pores; however, despite synchronous contraction of cardiac cells, macroscopic in-plane compression was not achieved [89–91]. Reducing the strut widths can increase scaffold compressibility, although the resulting increase in pore size must also be balanced with submicron-sized structures to guide cell alignment without compromising compressibility overall. Although contractile strength studies of engineered cardiac muscle tissues are rare, a few studies have found that cellular alignment (i.e., anisotropically aligned in comparison with isotropic) in thin films of cardiomyocytes increases contractile strength >1000% [53] and can increase twitch force up to 180% [61]. Peak systolic stresses in these anisotropically aligned films can reach >10 kPa when stretched at 0.5–5.0 Hz, in comparison with isotropic films that only reached peak systolic stresses around 1 kPa at 0.5 Hz and did not fully relax during the contractile cycle when stretched at >1.0 Hz [53].

Hydrogels, which can have different cross-linking densities and compositions to control substrate stiffness that in turn influences cell morphology and phenotype, encompasses a large portion of the literature on cardiovascular biomaterials; however, applying spatial cues to hydrogels still requires micropatterning to apply biomolecules (discussed in a later section) or lithography techniques (e.g., applying masks during photopolymerization or using molds for shaping). For an in-depth review specific to spatiotemporal control of hydrogels, we refer to the recent publication from Kharkar and colleagues [92].
To compensate for mechanical strength while still accommodating contractility, electrospun nanofibers can recreate topological cues on the same length scale as native ECM to encourage cellular self-assembly along 3-D fiber-like elements [63,93,94]. Electrospinning allows many parameters to be manipulated, including fiber orientation, density, and length; moreover, synthetic polymers, natural ECM, and polymer blends can be electrospun. Suspended nonwoven unidirectional nanofibers packed at 20- to 30-μm spacing provided enough guidance and structural support to grow a continuous anisotropic tissue using cardiomyocytes; tissue stripes and gaps in the tissue formed instead at greater packing distances [93]. Similarly, SMCs orient along fiber lengths on electrospun fabrics [95].
Decellularization uses combinations of mechanical means, detergents, enzyme inhibitors, and buffers to treat natural tissues, usually allogeneic [96–98] or xenogeneic [99–102] in origin. Cells are stripped to (a) passivate antigenic epitopes and (b) leave behind natural ECM for its biocompatibility and mechanical properties [14]. As shown in biaxial mechanical tests separating tissue layers consisting of mostly collagen fibers under tension, anisotropic materials can be separated longitudinally and isotropic materials can be separated circumferentially; however, the processing methods for removing cellular material can alter fiber alignment and matrix integrity, thus changing the mechanical behavior of the resulting scaffolds [103,104]. In addition, decellularized tissue scaffolds can be reseeded with autologous differentiated cells to further reduce immunogenicity [97,105]. However, the tight matrix structure usually makes cell migration difficult during recellularization [14,100], and the treatment process itself may cause structural alterations [103,106,107]. Acellularized myocardial scaffolds have shown stiffer mechanical behavior that may be restored with recellularization [108–110]. For vascular tissue engineering, human umbilical veins [111,112], coronary arteries [113], urinary bladder [114], and small intestinal submucosa (SIS) [21,115] have been used as decellularized scaffolds, with similar difficulties in cell infiltration. Clinical use of decellularized tissues remains limited, with efforts focused on in vitro or accelerated in situ with autologous cells [14,112]. A methods comparison of whole organ decellularization [112] and the structure and function of intact decellularized tissues [103] have been reviewed in-depth elsewhere.
13.3.2 Bioreactors and Bioactive Molecules: Active Exogenous Forces
Although tissue engineers have made great strides forward, culture times for many current constructs simply are still too long (≥1 month) [116–119], which increases risk of contamination, or the cell may change phenotype [65,120,121] or dedifferentiate [118,122–126]. Inappropriate or absent stimulations may cause cells to become disorganized and apoptose [63]. Tissue engineers try to simulate native physiologic conditions to elicit desired cellular remodeling responses in vitro, and adding an exogenous cell signal to a construct encourages accelerated ECM production, maturation, and integration of engineered tissues [39,127–130], which generally fall into three categories:
- Biochemical Cues. Scaffolding surfaces can be functionalized with bioactive or bioadhesive molecules with methods such as microcontact printing (μCP). Additionally, the culture environment can be supplemented with growth factors and other chemokines.
- Electrical Fields. Cardiac contractions are propagated by electrical signals, to which any grafts must synchronize, or arrhythmias may occur. Electric fields have also been naturally detected in skin wounds, damaged tissues, and vasculature, which may be useful in directing migration of cells for repair and could be exploited for tissue engineering constructs [131].
- Mechanical Conditioning (Bioreactors). Cardiovascular cells are subjected to constant mechanical forces (e.g., cyclic deformation, pulsatile flow, fluid shear) that are necessary to direct blood flow. However, injured tissues may have undesired stress environments that negatively impact remodeling (e.g., cardiac dilatation, atherosclerotic lesions), such as disarranging cells essential for effective contractions, or releasing (or lack thereof) autocrine and paracrine factors [132–135].
The number of studies dedicated to determining the optimal combination of growth factors and other bioactive molecules to produce desired behavior (e.g., increase ECM secretion, increase yield of preferentially differentiated cells) is abundant. Tissue engineers also modify scaffolds to control drug release, immobilize angiogenic growth factors and other ligands, or engineer chemoattractant gradients to encourage cell adhesion and migration. We focus our discussion here on microfabrication, which can be used to micropattern scaffolds and substrates with bioadhesive molecules or ECM proteins that allow for temporal or spatial control of bioactive molecules to increase alignment or improve other hierarchical structures (Figure 13.4) [136]. Biomolecules can be applied by either restricting or stamping the area intended to be activated.

Soft lithography techniques can be used to create microfluidic channels or stencils for restricting the delivery of bioactive molecules and cells (Figure 13.4A) [136]. Masks with microchannels or cutouts are formed using PDMS, which are then self-sealed on top of the substrate. Suspensions of cells or biomolecules can then be (a) streamed through the microchannels using laminar flow or (b) added directly to the exposed stencil cutout areas for attachment before removing the mask. The masks allow for spatial control, but diffusion of small molecules and leakage of cells can occur using these blocking methods.
μCP allows patterned shapes that resemble native architectures to be created, giving precise means to apply spatial cell adhesion cues to surfaces (Figure 13.4B) [85,137]. A precisely patterned stamp (usually PDMS) is generated using soft lithography techniques that can then be inked or coated with bioactive molecules or ECM proteins and applied to a surface using conformal contact. Because PDMS is hydrophobic and DNA and other biomolecules are hydrophilic, the stamp can undergo oxygen plasma treatment, chemical treatment (usually silanes), or adsorption of polar molecules (e.g., positively-charged dendrimers) to allow homogenous inking with hydrophilic molecules [138]. Resolution and feature definition by μCP are limited to the mechanical properties of the stamp, and any deformities the stamp (e.g., buckling) may undergo during stamping may also be observed on the stamped substrate. Hybrid copolymers that allow for stiffer molds may be used for creating features on the nanoscale or with higher relief aspect ratios [139]. Additionally, most stamps only ink one molecule at a time, although creating a stamp with a microfluidic network to allow simultaneous inking of several molecules has been suggested [140]. Similarly, affinity and other supramolecular interactions (e.g., target and ligand molecules) can be used in affinity contact printing (αCP) (Figure 13.4C), but with the added advantage of reversible interactions through the complementary ligand units, which are usually immobilized on the stamp, so that stamps can be reused [138,140,141]. αCP allows for simultaneous capture of different molecules (e.g., DNA, antibodies) from a heterogeneous solution and easy inking for repeated printing, but the interaction between the target molecule and the ligand must be weaker than the interaction between the ligand and the stamp. μCP is more commonly used for cardiovascular applications, such as patterning fibronectin on substrates to recreate the aligned striations of cardiac muscle [142] or SMCs for vascular patches [143].
Electric fields can also be used to increase the structural organization of cardiovascular cells in tissue-engineered constructs. Applying synchronous contractions to cardiomyocytes induces preferred structural alignment in direction of applied electric field, which directly translates to higher average conduction velocity in comparison with nonstimulated tissues [144]. Cardiomyocyte alignment reduces the excitation threshold voltage and increases the amplitude of contraction, as aligned cells were shown to propagate signal along the longitudinal direction faster than in the transverse direction [53,145]. Additionally, contractile protein (troponin I, myosin heavy chain, sarcomeric α-actin) and gap junction protein connexin-43 (Cx43) expression increased in aligned cells [146,147]. Distribution of Cx43 expression has been shown to change significantly in cardiac diseases that lead to arrhythmias [148–150].
Although obvious for cardiac tissue, applying electrical fields also has potential in vascular tissue engineering, as endothelial progenitor cells (EPCs) have responded similarly with elongation and alignment [131]. Inhibiting the vascular endothelial growth factor (VEGF) receptor completely stopped electric field-induced migration of EPCs. Similarly, pulsatile electrical stimulation has been used in scaffolding made from the electroresponsive polymer polyacrylic acid to allow cells to infiltrate the scaffolding and induce alignment of SMCs [151]. To a lesser extent, magnetic fields have also been applied to hydrogels embedded with magnetic wires to induce collagen orientation in vascular grafts [152] or changes to cell morphology [153].
In addition to improving construct size, cellularity, and molecular composition [39,154–156], bioreactors can be used to enhance cellular anisotropy and mechanical behavior under specific hydrodynamic conditions [77] and physical stimuli, such as dynamic compression and cyclic stretch [157–160]. Multiple bioreactor devices have been made that apply tensile loading, compressive loading, and convective flow to study biologic responses and engineer tissues, which are described in detail elsewhere [161–163]. Because compressive systems are generally nondirectional in applying hydrostatic pressure, the focus on applying physiologic mechanical stimulation to bring structural hierarchy to cardiovascular tissue engineering constructs has been on cyclic tension, fluid shear, and hydrodynamic forces.
The application of uniaxial cyclic tension to cardiovascular cells (cardiomyocytes, ECs, SMCs) has shown repeatedly that the cells self-organize and elongate in an orientation perpendicular to the stretch axis in the absence of a scaffold [127,164–171]. This alignment may allow for coordinating wave-like contraction propagation and for absorbing deforming forces from contraction. As with electric fields, applying stretch has shown increased anisotropic distribution of Cx43 [166,172]. Furthermore, uniaxial cyclic tension applied longitudinally to an ECM scaffold with active cellular remodeling will further align fibers, especially collagen, along the stretching direction [173–176]. Again, the evidence indicates that scaffolds provide important architectural foundation cues via contact guidance, as cells and mechanical stimuli work synergistically to remodel the matrix.
Rotary suspension cultures and spinner flasks have been used to apply fluid shear and hydrodynamic forces on pluripotent stem cells to (a) control aggregation into embryoid bodies (EBs) and (b) accelerate differentiation into cardiovascular cells [177], especially cardiomyocytes [178–186] and ECs [187–191]. Suspension cultures allow for size-controllable scale-up production of EBs over tedious methods like hanging drop formation and other static suspension cultures that produce heterogeneous EB sizes and, thus, heterogeneous differentiation and low yields [192–194]. Fluid shear has also been used in maturation of larger 3-D constructs, controlling the size of scaffold-free cardiac patch constructions [195,196], discussed in the section below.
Convective flow from perfusion bioreactors has been used to homogenize initial cell seeding densities and precondition vascular grafts using pulsatile flow [39,63,116,119,197–199]. Because the functionality of ECs correlates with shear stress, engineered vessels are often flowed under physiologic pulsatile conditions to line the lumens with ECs. The physiologic flow conditions also modulate collagen remodeling in tissue-engineered blood vessels [198]. Additionally, constructs cultured under pulsatile flow had tissues that were twice as thick as those under constant flow, and resulting burst strengths were an order of magnitude higher for small-caliber grafts seeded with various cell types [116,119]. Increased alignment of cells and collagen were also observed in constructs under pulsatile flow for over a month, while constructs under constant flow also demonstrated dedifferentiated cell phenotypes.
Although exogenous cues greatly enhance tissue maturation, contact guidance using topographical cues, as discussed in the previous section, has still been demonstrated in numerous studies to have a greater effect on cellular alignment cues. For example, in studies comparing the interactive effects of contact guidance and electrical stimulation, cardiomyocytes and cardiac fibroblasts preferentially oriented along topographical alignment cues, even when electric fields were applied perpendicular to the grooves [200–202]. When electrical stimulation fields were applied parallel to topographically aligned cells, the effect was additive in elongating cells. Additionally, smaller spatial cues (e.g., 1- to 10-μm grooves) have a greater effect on cell elongation, as focal adhesion protein vinculin was observed to be localized along the grooves [201], and larger grooves may yield less functional tissues as gap junction formation between cells for cellular communication is impeded [203]. A similar study comparing the interactive effects of contact guidance and mechanical conditioning found similar results, in which mechanical stretch was applied to cells aligned perpendicular or parallel to the stretch direction in microgrooves [204]. Unconstrained cells that are mechanically stimulated normally orient perpendicular to the direction of stretch; cells previously aligned within grooves perpendicular to the applied strain showed a significant increase in the strength of cellular alignment. However, cells constrained within microgrooves parallel to the strain direction tended to stay aligned in the groove direction, suggesting that the microtopographical cue has a greater effect than mechanical stimulation. Cells seeded within 70-μm grooves were able to demonstrate some reorientation to cyclic strain, in which the width of the groove may have been wide enough for cells to maneuver.
13.3.3 Cell Sources: Cell Phenotype and Secreted Extracellular Matrix
To truly restore normal function, tissue engineering in regenerative medicine must incorporate the cell—whether in vivo, ex vivo, or in vitro
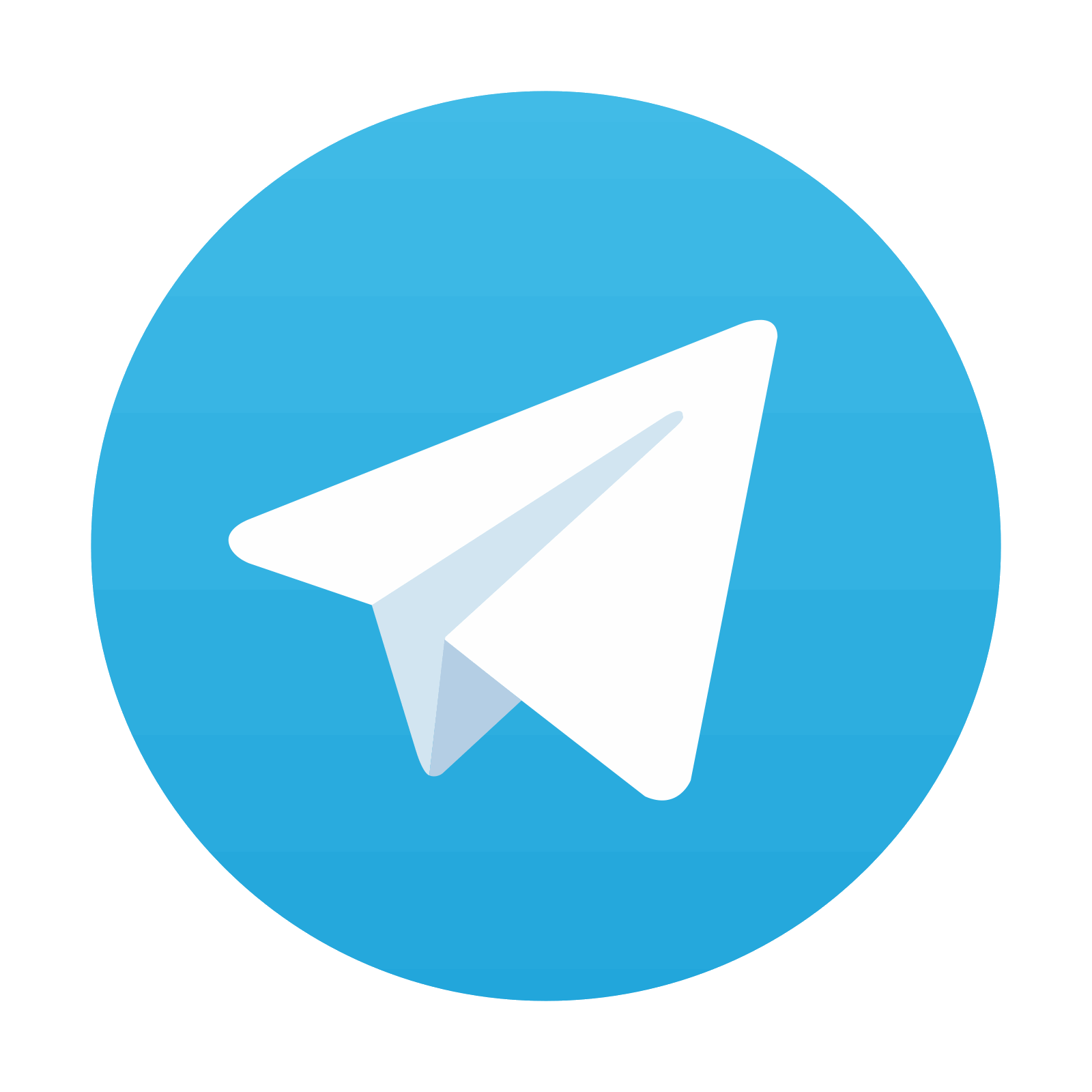
Stay updated, free articles. Join our Telegram channel
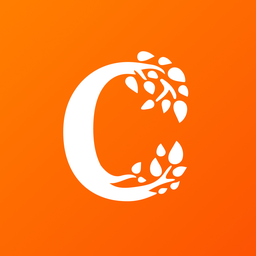
Full access? Get Clinical Tree
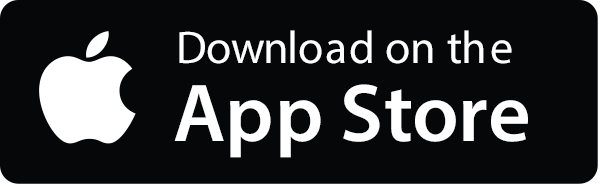
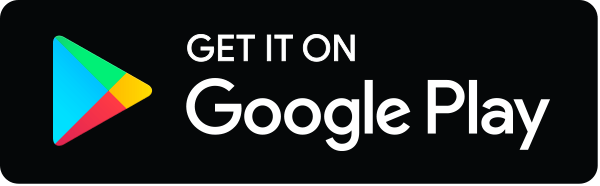