Nano- and Microstructured ECM and Biomimetic Scaffolds for Cardiac Tissue Engineering
Department of Biomedical Engineering, Carnegie Mellon University, Pittsburgh, PA, USA
Department of Biomedical Engineering, Department of Materials Science and Engineering, Carnegie Mellon University, Pittsburgh, PA, USA
12.1 Introduction
Cardiovascular disease (CVD) has become the principal cause of death in the developed world, causing one in three US deaths in 2009 (787,931 total) [1]. The most common form of CVD is coronary heart disease, where the coronary vasculature supplying the heart tissue with oxygen and nutrients becomes occluded. When starved of oxygen for too long, it causes a heart attack, or myocardial infarction (MI), where the cardiomyocytes (CMs) die and are unable to regenerate. MI was responsible for one in six US deaths in 2009, and associated health care costs amounted to $195.2 billion [1]. This demonstrates the increasing burden of CVD on the society. Moreover, patient morbidity leads to a considerable decrease in their standard of living. To address this issue, significant basic and clinical research efforts are focused on the development of strategies to restore cardiac function.
Therapies to treat MI and heart failure face unique challenges, chief among them is the absence of natural regeneration in adult cardiac tissue. CM proliferation, important during development, nearly disappears after birth [2]. Instead, postnatal myocardial growth is mostly due to CM maturation and enlargement, termed hypertrophy, which can bring a 20-fold increase in cell size [3]. Recent findings suggest that there is a basal level of CM proliferation even in adults, but fewer than 1% are replaced every year, meaning more than 50% of CMs are sustained throughout our lifetime [4]. After injury, the percentage of CM proliferation near the infarct site rises to 3%, but it is insufficient to naturally recover cardiac function [5]. Damaged myocardium is then further remodeled during the acute inflammatory response and is eventually replaced by hypoxic and noncontractile scar tissue. Therapeutic approaches aim to recover full or partial cardiac function, either by inducing the formation of viable cardiac tissue or by compensating for the damaged tissue with medical devices.
With disease progression or recurrent MI, patients eventually suffer heart failure, characterized by the inability to pump enough blood to the body. A heart transplant is the gold standard for treatment, and while it is estimated that 40,000 patients could benefit, there are only about 2200 donor hearts available each year [6]. To address the urgent need for circulatory assist, devices such as total artificial hearts and left ventricular assist devices have been developed [7]. These mechanical pumps work short term, but have serious limitations as they can trigger thrombosis, leading to blood clots that circulate to the brain or the lungs, block small arteries, and induce stroke or embolism. Furthermore, excessive shear stress within these mechanical assist devices can damage the blood cells, causing hemolysis. Thus, survival is improved only for ∼5 years, with these devices used predominantly for bridge to transplantation instead of destination therapy for heart failure [8]. More recently, stem cells have been used in an attempt to regenerate and repair damaged heart tissue [9–13]. Stem cell therapy can effectively modulate the effects of the acute inflammatory response after a heart attack via the release of paracrine factors that reduce CM death and preserve functional myocardium that would otherwise be remodeled to form scar tissue [11]. However, long-term outcomes are hindered by the inability to recover the bulk of cardiac muscle lost during MI. More than 90% of stem cells injected into the infarct die after a week in the hostile environment with little to no new muscle formation or functional integration [11]. Additionally, exogenous cells introduced into the complex cardiac environment have the potential to create arrhythmia due to inadequate electromechanical coupling [14].
Cardiac tissue engineering has emerged as a promising solution to address these challenges and produce functional cardiac tissue. While approaches vary, typically, polymer scaffolds are engineered to provide physical and chemical cues that guide the behavior of cardiac cells and instruct them to proliferate, differentiate, and eventually assemble into a viable tissue. The primary goal is to develop a construct with dense, anisotropic cardiac muscle that has electromechanical function on par with ventricular myocardium. The ultimate tissue engineering application is to repair an MI in a patient, by either replacing the damaged tissue with an in vitro-engineered cardiac muscle graft or implanting a scaffold in vivo that promotes endogenous repair. Additionally, engineered cardiac muscle has great utility as an in vitro model system of cardiac function, enabling researchers to test the effects of drugs for safety and toxicity, as well as discover new compounds to treat a range of CVD. To inform scaffold design, cardiac tissue engineering draws from cardiac anatomy and physiology, advances in stem cell biology and, most importantly, from a better understanding of the heart’s native scaffold, the extracellular matrix (ECM). The ECM is essential to cardiac structure and function and it helps provide and transduce the physical and chemical cues required by cardiac cells to maintain tissue homeostasis. For these reasons, researchers have developed a range of technologies to engineer scaffolds that mimic the cardiac ECM in order to achieve the production of functional cardiac constructs. Cardiac tissue engineering, by using bio-inspired scaffolds to induce tissue growth, has the potential to treat CVD in millions of patients every year.
In this chapter, we provide an overview of the heart and the biomimetic approaches used to engineer cardiac muscle tissue. We first describe the unique structure and function of cardiac muscle (myocardium), discuss how this dictates scaffold design, and then define the benchmarks used to evaluate the performance of engineered cardiac tissue. Next, we present the different techniques researchers have developed to fabricate tissue-engineered cardiac scaffolds that mimic ECM cues observed in the heart. For each case, we discuss the relevant physical, mechanical and/or chemical properties and the particular advantages and limitations of the approach. Finally, we discuss the persistent challenges to engineering functional cardiac tissue and the future directions of the field.
12.2 Structure and Function of the Myocardium
12.2.1 Multiscale Hierarchy of the Contractile Apparatus
The myocardium features a complex hierarchical organization that spans from the molecular to tissue scales, encompassing eight orders of spatial magnitude (Figure 12.1). At the nanometer scale, contractile forces rely on the interaction of molecular motors composed of actin and myosin filaments organized into overlapping bands that form the basic contractile unit termed the sarcomere. Sarcomeres assemble into cytoskeletal filaments termed myofibrils that span the entire CM cell body, and myofibrils within CMs bundle in parallel to form aligned contractile structures. The 2–4 billion CMs in the heart are cylindrical in shape, with a length to width aspect ratio of 7 : 1, and are longitudinally connected end to end by specialized structures, termed intercalated disks, that mechanically and electrically couple the cells into multicellular, contractile myofibers [3,15]. At the tissue scale, the heart consists of lamellar-like layers of aligned cardiac myofibers wrapped around the heart to form the walls of the ventricles and the atria. In the left ventricle, which sends the blood to the body through the systemic circulation, myofiber orientation varies linearly throughout the thickness of the ventricular wall (Figure 12.2). This architecture is responsible for translating the uniaxial contraction of individual cells into an actual reduction in the volume of the heart chambers during a pumping cycle. Thus, it is the coordinated action of billions of actin–myosin molecular motors at the nanometer scale, each generating piconewton forces, that produces tissue-scale forces up to 10 mN/mm2 of myocardium [16,17]. Engineered cardiac constructs need to reproduce the complex hierarchical structure of the myocardium in order to provide clinically relevant contractile forces.


12.2.2 Mechanical Anisotropy
The complex arrangement of aligned myofibers gives the myocardium highly anisotropic mechanical properties. For example, the native myocardium of the left ventricle of a rat has a Young’s modulus of 157 ± 84 kPa parallel and 84 ± 8 kPa perpendicular to the myofibers (Figure 12.3), with similar values reported for human and porcine hearts [18–22]. The importance of the mechanical properties and anisotropy is illustrated by the profound alterations observed in disease states. In a rat model of MI, ventricular stiffness increased twofold at 6 weeks after the MI, while the scar tissue within the MI became completely isotropic in mechanical properties [23]. In vitro, CMs cultured on substrates stiffer than normal myocardium have demonstrated a decreased beating frequency, further suggesting that ventricular stiffening can adversely affect CM contraction and cardiac function [24]. This indicates that in order to achieve therapeutic success, scaffolds for cardiac tissue engineering must match the unique mechanical properties of the native myocardium. Specifically, CMs contract ∼10% during the cardiac cycle in order to empty the ventricle and eject blood. Achieving this deformation in an engineered cardiac tissue requires that the effective elastic modulus of the combined cells and scaffold be closely matched to the native myocardium.

12.2.3 Innervation and the Conduction System
The heart has a complex control system to regulate heart rate and the contraction cycle, and like every muscle, the heart is densely innervated. Heart rate and cardiac output are influenced by a network of afferent and efferent neurons that extend to the brain and the spinal cord, as well as interconnected neurons within the heart [25]. However, direct control of heart rate originates in the sinoatrial (SA) node, a group of cells in the right atria that initiate each contraction of the heart by regularly and autonomously depolarizing (Figure 12.4) [26,27]. Electrical signals generated in the SA node propagate as an action potential through the myocardium via the gap junctions, which are intercellular ion channels that transmit electrical potentials via Ca2+ flux. The arrangement of CMs in aligned myofibers accounts for the anisotropic electrical properties, with faster action potential propagation longitudinally than transverse [28]. From the atria, the action potential reaches the atrioventricular (AV) node, which connects to a ring of CMs that loops between the ventricles and the atria. The AV node slows conduction by introducing a delay to ensure enough time for the atria to empty into the ventricles. The action potential is then transmitted to the ventricular conduction system, which comprises the Purkinje fibers. The specialized CMs of the Purkinje fibers have higher conduction speed in order to rapidly distribute the action potential throughout the ventricles so that they can contract synchronously. Together, the nerves, nodes, and ventricular conduction system coordinate and regulate contraction to meet systemic needs. However, disease states can disrupt normal contraction and cause an arrhythmia, which can be fatal. Loss of synchrony, whether due to genetic defect or tissue remodeling after MI, prevents coordinated contraction of the ventricles, thus reducing or eliminating blood flow [25,29,30]. For cardiac tissue engineering, grafts intended to replace infarcted scar tissue must integrate successfully with the native neuronal and conduction systems and synchronize with the viable myocardium in order to improve cardiac function. Failure to do so will create a pro-arrhythmic interface between the graft and the patient’s myocardium. It remains to be determined how difficult it is to achieve this integration, as there are no clinical trials of engineered tissue grafts that have yet been conducted in humans.

12.2.4 Vascularization
The CMs in the heart are constantly contracting, creating a high metabolic demand that requires a large supply of oxygen and nutrients. To meet this need, the heart is the most vascularized organ in the body with capillaries spaced ∼20 μm, which maximizes mass transport to and from the CMs [31]. The coronary vasculature forms an extremely intricate vessel network branching throughout the myocardium from the ∼6 mm diameter coronary artery to the ∼10 μm diameter capillaries (Figure 12.5). The high capillary density means each CM is in contact with endothelial cells from at least one capillary [32]. While endothelial cells line the capillaries, larger vessels are supported by pericytes and smooth muscle cells that line the outer perimeter and maintain vessel integrity and vasoactivity. These vascular cells represent a significant fraction of the total number of cells in the heart and have an essential role in regulating CM function. In the context of cardiac tissue engineering, tissues must have sufficient CM density to generate contractile force and high capillary density to provide adequate nutrient transport in three-dimensional (3-D) constructs. For example, cell density of more than 5 × 105 cells/mm3 is observed in the adult rat myocardium, of which ∼17% are cardiac vascular cells (endothelial cells and pericytes) [33,34]. The cardiac endothelium, in particular, can regulate cardiac function via paracrine signaling between endothelial cells and CMs [35,36]. For example, when cultured with endothelial cells in vitro, CMs showed improved survival rate and contractile performance [37]. In cardiac tissue engineering, it is important to account for the coronary vascular system in terms of nutrient mass transport to highly metabolic CMs, as well as specific cardiac endothelium–CM signaling. Recapitulating both aspects is likely necessary in order to achieve clinically relevant CM density and function.

12.2.5 Extracellular Matrix
The cardiac ECM is a 3-D network of fibrillar proteins and glycosaminoglycans (GAGs) that provides structural support and serves as an essential substrate for physical and chemical signaling. Each cell type in the heart contributes in some way to the synthesis, assembly, and remodeling of the ECM in their local environment. However, cardiac fibroblasts are the primary cell type involved in the maintenance of the ECM and represent 64% of the total number of cells in the adult rat heart, though they account for only ∼18% of the cardiac volume [33,38]. Cells interact with the ECM through integrin receptors on their membrane that can bind to the ECM and form a mechanical linkage to the cytoskeleton within the cell. Through this binding interaction, cells can exert mechanical forces on the ECM to migrate through their environment or to manipulate and assemble the surrounding ECM. In order to remodel the ECM, cells also release specialized enzymes, the matrix metalloproteinases (MMPs), which can cleave specific ECM components [39].
There are a large number of proteins within the cardiac ECM that contribute to its structural, mechanical, and chemical properties. The first element of the ECM interacting directly with the CMs is the basement membrane, a thin protein layer composed mostly of laminin, fibronectin, collagen type IV, and perlecan, a basement membrane-specific heparan sulfate [40,41]. The basement membrane acts as a selective barrier for soluble factors, as well as a linkage to the structural fibers of the extracellular environment. Beyond the basement membrane, collagens type I and III provide tensile strength and maintain the shape of the heart while also distributing the contractile forces to the whole organ [42–44]. They form a complex weave that surrounds bundles of adjacent CMs and long coiled strands aligned with the main myofiber orientation [45]. Each CM is tethered to the collagen matrix by costameres, protein assemblies that link the sarcomeres to integrin receptors to the ECM, which prevent slippage or injury under excessive loading [44,46]. Additionally, the myocardium contains the structural fiber elastin, a cross-linked, flexible protein that is highly expressed in blood vessel walls and contributes to the heart’s elasticity [20]. Structurally, the cardiac ECM has characteristics of the fibrillar and laminar components, featuring an interconnected network of small pores, organized very tightly around cardiac cells. For example, the average pore diameter was 21.4 μm in decellularized porcine myocardium (Figure 12.6) [20]. The fibrillar proteins also exhibit a wide range of diameters, ranging from ∼1 μm for elastin, from 10 nm to 10 μm for collagen type I, and from 5 nm to 1 μm for fibronectin [47–49].

The importance of the ECM in normal cardiac function is highlighted by the maladaptive changes that occur in structure and composition with disease and aging. For example, the ECM collagen content and the diameter of the collagen fibers increase with age. This contributes to loss of elasticity (i.e., increase in stiffness) and is associated with a higher risk of CVD [42]. Furthermore, abnormal turnover of ECM proteins such as collagens, fibronectins, and laminins due to increased MMP activity is observed in many cardiomyopathies [50]. The ECM is also important in wound healing in the heart. During the acute phase of MI, the normal cardiac ECM is replaced by a provisional fibrin-based matrix. In the chronic phase, a dense, collagen type I-rich scar tissue is formed that is stiffer and has lost the mechanical anisotropy of the healthy tissue, which further hinders cardiac contraction [23,51]. Thus, the cardiac ECM is a key model system for designing cardiac tissue engineering scaffolds, and further highlights the need to recapitulate properties of the healthy ECM in order to produce viable cardiac constructs.
12.3 Bio-inspired Design Requirements of Cardiac Tissue Engineering Scaffolds
The structure and function of the myocardium as highlighted in Section 12.1 forms the basis from which we can implement the bio-inspired design of cardiac tissue engineering scaffolds. While the myocardium contains complex contractile, electrical, and vascular networks, it is the cardiac ECM that integrates these together into a functional tissue system. Thus, it is the cardiac ECM that many researchers look to as a design template for building biomimetic scaffolds. Significant research has focused on the structural, mechanical, and biochemical characteristics of the ECM and methods to recapitulate a subset of these properties using synthetic or naturally derived polymer scaffolds.
Porosity is a key component of scaffolds because CMs need to be able to infiltrate and couple together into a dense network to form functional myocardium, requiring a network of interconnected pores. In scaffolds that cannot be easily remodeled by the cells, the absolute minimum size for pores is the size of a cell, which based on measurements of the decellularized myocardium is ∼20 μm [20]. Because the myocardium is cell dense, the scaffold must have high porosity or be able to be resorbed or remodeled by the cells to achieve a high porosity. Based on the ECM density in the heart, this requires a scaffold porosity of >90% to sustain high cell density, ensure homogenous cell seeding, and support oxygen and nutrients mass transport essential to the function of cardiac tissue. In the case of low porosity or very small pore size, specialized techniques such as the use of medium perfusion, are required for efficient cell seeding and oxygen and nutrients delivery to the bulk of the scaffold.
The cardiac ECM is composed of protein fibers such as collagen type I and III, fibronectin, and elastin, which are organized in parallel with the myofibers and provide alignment cues to the constituent CMs. It is important to recapitulate the structural characteristics of these fibers because CMs, like all cells, respond to physical structures in the nanometer to micrometer range via a contact guidance effect. The key physical attributes of ECM fibers are the diameter (typically ranging from 5 nm to 10 μm), spatial density, and 3-D orientation. Thus, scaffolds with fibers or fiber-like features that mimic the structure of the cardiac ECM can be effective for the alignment of CMs and other cardiac cells into anisotropic tissues.
In the myocardium, cardiac cells are tightly connected to the ECM via integrin receptors, and this adhesive binding is necessary for survival and function. For example, disruption of fibronectin binding, observed in mice without the integrin α5β1, is embryonically lethal [52]. The specific ECM protein composition and organization is also important, as fibronectin, which is expressed at higher levels during cardiac development, has been shown to foster cardiac progenitor cell differentiation into CMs [53]. Cardiac tissue engineering scaffolds composed of ECM proteins typically have intrinsic ligands for integrin receptors to bind cells, comparable with the native ECM. However, synthetic materials, polysaccharides, and other non-ECM protein materials must be modified in some way to add cell adhesive motifs. Typically, this is achieved by grafting or adsorbing polypeptides or whole ECM proteins (such as fibronectin) to the materials as either a surface or bulk modification.
In the heart, cells remodel the cardiac ECM continuously to allow for myofiber formation, angiogenesis, and high cell density. For example, collagens are completely replaced every 160–240 days and noncollagen proteins are replaced 10 times as fast [54]. It is essential that cardiac tissue engineering scaffolds degrade in a controlled manner in order for cells to organize and maintain their own ECM. Scaffolds made from native components of the ECM can readily be remodeled by cells because they already express the appropriate enzymes, such as MMPs. For synthetic materials, remodeling can be achieved by using biodegradable polymers that cleave by hydrolysis and/or integrating enzyme-cleavable domains, comparable with the MMP-degradable sites in ECM proteins. Moreover, the kinetics of scaffold degradation should be coordinated with ECM production and remodeling to match the rate of muscle formation and maintain structural integrity throughout the process.
Finally, scaffolds need to integrate with the electrical and mechanical properties of the cardiac environment. For example, constructs must exhibit high fatigue resistance by reversibly withstanding mechanical strains of ∼13% over a large number of contraction–relaxation cycles, millions if grafted in the actual heart [55,56]. Scaffolds also need to match the mechanical compliance and anisotropy of the myocardium to promote CM alignment and contractility. Based on rat and human myocardium, the Young’s modulus should be in the range of 10 kPa to 1 MPa and be higher in the myofiber direction than transverse. The CMs must also be aligned uniaxially within each lamellar layer, for example 50% of the CMs in the rat right ventricle are aligned within ±20° of the mean alignment direction [18]. Furthermore, scaffolds must not negatively impact the electrical properties of cardiac tissue, in particular, it must have similar conduction velocity and anisotropy to native myocardium and the impedance should be low to avoid hindering contraction and causing necrotic/hypoxic myocardium [57].
12.4 Approaches to Fabricating ECM Biomimetic Scaffolds
12.4.1 Porous Scaffolds
Fabricating scaffolds with an interconnected network of pores is the most straightforward way to obtain a thick 3-D structure that can be seeded with cells throughout and sustain high cell density. For cardiac tissue engineering, porogen leaching and lyophilization are the methods that have produced the best results. In porogen leaching, a polymer solution is mixed with a porogen (e.g., salts or sugars) and polymerized. The porogen is then dissolved using a solvent, which creates voids within the polymer matrix. By controlling the size and density of porogen particles, scaffolds can be engineered with pore sizes ranging from one to hundreds of micrometers and porosities of up to 95% of the scaffold volume [58]. For example, Radisic et al. used sodium chloride (NaCl) particles as porogens to fabricate poly(glycerol sebacate) (PGS) scaffolds with interconnected pores between 75 and 150 μm in diameter and 90% porosity (Figure 12.7A) [59]. These scaffolds allowed seeding of CMs and cardiac fibroblasts at high density in vitro and when implanted in a mice model of MI, acellular porous PGS scaffolds were readily invaded by host cells and supported the formation of new blood vessels [59,60]. Porogen leaching also can be adapted to provide more control over the macroscale architecture of the scaffold. For example, Madden et al. engineered porous scaffolds that combined regularly spaced polycarbonate (PC) rods and poly(methyl methacrylate) (PMMA) beads in order to create an array of parallel channels in the poly(2-hydroxyethyl methacrylate-co-methacrylic acid) (pHEMA-co-MAA) hydrogel scaffold (Figure 12.7B) [61]. The result was a high-porosity scaffold with an array of 60-μm wide channels in place of the rods that fostered CM bundle formation and 30-μm pores in place of the beads that improved neovascularization in an infarct model.

Lyophilization, or freeze-drying, is another method for creating porous scaffolds, and is most commonly used for naturally derived biopolymers. To do this, a polymer dissolved in water is frozen and then exposed to near vacuum to sublimate the ice crystals that formed during the freezing process, leaving behind pores. This technique can be used with polysaccharides such as alginate to produce scaffolds with tunable pore size and mechanical properties (Figure 12.7C) [62]. Furthermore, polysaccharide scaffolds can be chemically modified with cell-adhesive proteins found in the ECM. For example, alginate scaffolds with ∼100-μm pores fabricated by lyophilization were modified with the integrin-binding peptide arginine–glycine–aspartic acid (RGD), and heparin-binding protein (HBP) [63]. Culturing CMs on the alginate–RGD–HBP scaffolds resulted in increased expression of sarcomeric α-actinin, indicating increased muscle volume compared with CMs cultured on nonmodified alginate scaffolds. Additionally, lyophilization can be used to engineer scaffolds with anisotropic pore structures by controlling thermal gradients during the freezing process. For example, when a collagen–GAG solution is frozen in a Teflon cylinder between two copper plates, the difference in thermal conductivity between copper and Teflon creates a unidirectional pore structure. After freeze-drying, these scaffolds had elongated pores up to 243 μm in diameter, with an aspect ratio of up to 12.6 : 1, which promoted alignment and beating of the human CM cell line HL-1 in vitro (Figure 12.7D) [64].
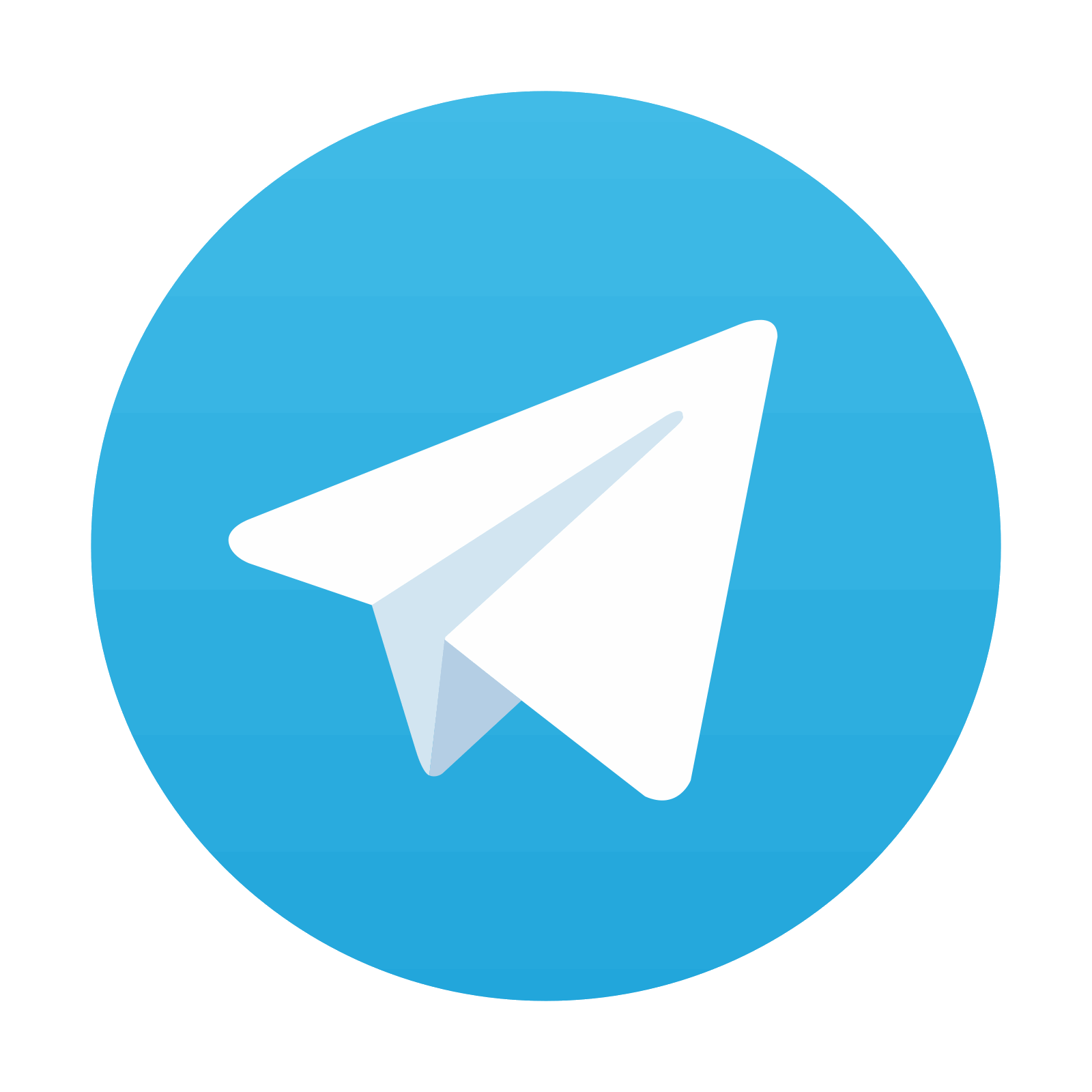
Stay updated, free articles. Join our Telegram channel
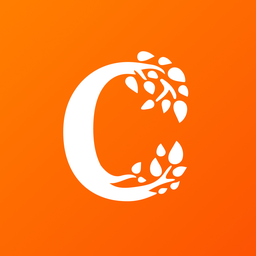
Full access? Get Clinical Tree
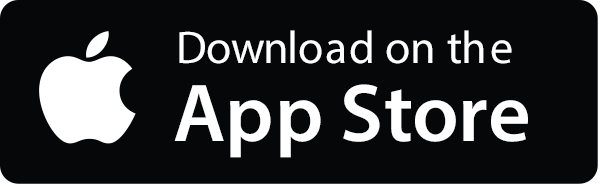
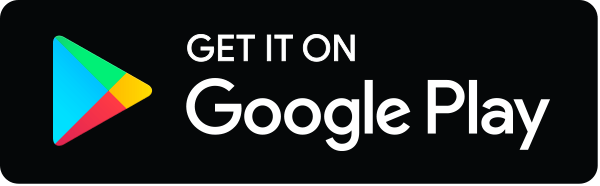