A Biomimetic Approach toward the Fabrication of Epithelial-like Tissue
Department of Chemistry, Chemical Biology and Biomedical Engineering, Stevens Institute of Technology, Hoboken, NJ, USA
11.1 Introduction
The skin is the largest organ and covers the entire exterior surface of the body. It plays a crucial role in maintaining the homeostasis of internal tissues and organs, keeping the balance of moisture and temperature. The skin also serves as an efficacious barrier against hostile attack from the external environment and protects the body from mechanical, osmotic, and thermal damage. The skin contains three primary layers: epidermis, dermis, and subcutaneous fat. The epidermis is waterproof and acts as a barrier to infection. The dermis provides support to the epidermis and accommodates various appendages of the skin. The subcutaneous fat serves as padding and insulation for the body, in addition to storing energy and supplying oxygen via the blood vessels. With constant exposure to the external environment, damage to the skin cannot be avoided, and in the worst case, it can threaten the human life. The skin has a remarkable ability to regenerate in the case of injury to the epidermal layer or the superficial region of the dermis; however, when the injury is deep, especially with a complete devastation of the hair follicles, the damaged skin cannot spontaneously regenerate. In this regard, massive deep skin loss can lead to acute physiologic imbalance and ultimately, cause significant disability or even death. It becomes essential to properly close the wounded area with an effective dressing or with skin grafts as soon as possible to prevent fluid loss and potential infection and to facilitate wound healing with skin regeneration.
Skin wound healing is a complex process that involves the migration and proliferation of keratinocytes and fibroblasts and the synthesis and remodeling of new extracellular matrix (ECM). During this process, the cellular functions are synergistically regulated by various growth factors and cytokines, which are upregulated by the wounded environment [1] via corresponding cell membrane–bound receptors. To help the closure of hard-to-heal, deep wounds, several treatment options are available. Among them, split-thickness autologous skin grafting remains the gold standard. However, the limited availability of autografts along with the morbidity and pain associated with tissue harvesting sites can be a significant barrier for its application. The next best alternative to autografts is decellularized allografts, which provide a natural three-dimensional (3-D) ECM structure and basement membrane and are known for their role in promoting normal wound healing [2]. However, prolonged time is required for cells to migrate into an acellular matrix from surrounding tissues [3–5], which can significantly delay new tissue formation and reepithelialization. In recognition of the challenges of autografts and allografts, efforts have been made to create skin-like constructs via a tissue engineering approach. Currently, several tissue-engineered skin grafts are available for wound repair. Despite successful demonstration of their potential utility in wound repair, current commercial tissue-engineered grafts suffer from the following: (1) prolonged culture time to create the grafts (typically 2–3 weeks) [6], (2) low rate of acceptance by the host (40–60%, not clinically acceptable) [7], (3) poor handleability due to fragile mechanical durability, and (4) high cost associated with the use of skin grafts (e.g., annual medical cost of using Apligraf® [Organogenesis, Canton, MA] for an individual patient is approximately $20,000). Chronic wound patients frequently suffer from diseases such as diabetes and obesity [8]. Hence, it remains highly desirable to fabricate functional skin grafts for regenerating damaged skin tissues with full restoration of the lost biological functions, but at a low cost and within a clinically acceptable time windows (e.g., less than 2 weeks).
Nanofibrous matrices prepared from electrospinning can maximally recapture the dimension and morphology of native ECM and support the adhesion and growth of various cells, showing great potential in the field of skin tissue engineering. This chapter highlights how various nanofibrous matrices are prepared, and specific elaboration is made on the fabrication of nanofibrous scaffold for skin tissue regeneration.
11.2 Skin ECM and Its Function
Skin is comprised of several different cell types. Keratinocytes are the most common cell type in the epidermis and form the surface barrier layer. Melanocytes are found in the epidermis but mainly reside in the basal layer, providing color to the skin. Fibroblasts are the major cellular components of dermal layer, which provides strength and resilience to the skin (Figure 11.1). The interactions between skin cells and their surroundings, that is, the ECM, play a crucial role in regulating the normal functions of the skin. For example, epidermal keratinocytes rest on a thin, ultrafine fibrous membrane called the basement membrane, mainly composed of types IV and VII collagen and laminins. The basement membrane is the fusion of two laminae, the basal lamina and the reticular lamina. The primary function of the basement membrane is to anchor the epithelium to the dermis. It also acts as a mechanical barrier, preventing malignant cells from invading the deeper tissues. Since the basement membrane proteins have been found to accelerate differentiation of endothelial cells, it is also essential for angiogenesis. The basement membrane not only allows the anchorage of keratinocytes, but also regulates their phenotype via the interaction with cell membrane–bound integrins. Unlike the epidermis, in the dermis, dermal cells (e.g., fibroblasts) are embedded in a 3-D fibrous composite ECM consisting primarily of collagen (notably, type I collagen) and elastin fibers embedded in an amorphous matrix of mucopolysaccarides. The collagen is arranged in a planar array of highly undulated, wavy, and meandering fibers in all directions, thus allowing the skin to stretch but preventing overstretching. The collagen and elastin fibers may be cross-linked with ground substance and between themselves to provide mechanical stability to the skin [9].

11.3 Skin Tissue Engineering and Scaffold Design
The primary purpose of skin tissue engineering is to provide a temporary barrier, a dermal matrix, or a transfer mechanism to facilitate the regeneration of damaged skin by using synthetic or natural materials. On complete healing, these materials should eventually disappear from the healed skin, by way of biodegradation or replacement by newly formed tissue. It is of great benefit to combine the wound healing process and the cellular elements of living tissue with sophisticated biomaterials to produce living skin equivalents with sufficient size and desirable functions. Recently, a great deal of attention has been paid to the utilization of fibrous scaffolds for tissue formation mainly due to the dimensional and morphological similarity of the fibers to native ECM, which in turn could regulate cell attachment, migration, proliferation, and differentiation. Various fabrication techniques have been explored to address some potential challenges associated with the fabrication of complex and multifunctional fibrous substrates, including the creation of large pores to facilitate cell infiltration, controlled formation of nanometer-sized fibers, and incorporation of the spatial distribution of specific proteins for cell attachment and remodeling. The fibrous structure fabricated by these techniques can determine the shape and mechanical performance of tissues and provide the cells with instructive external cues to guide tissue formation. It also provides physical supports for cells to attach and to grow. To appropriately maintain cell phenotype during skin tissue engineering, it is desirable for the scaffold to recapitulate the major features of native ECM of skin on a multilevel scale, from the composition, morphology, and topography to spatial organization. We believe that nanofibrous scaffolds will be an ideal substrate to support skin tissue formation due to their similarity to ECM fibers in both dimension and morphology. Indeed, the advantages of nanofibrous scaffolds in promoting skin cell growth and maintaining proper phenotype have been demonstrated in a number of studies [10–13].
In the search for appropriate substrates to support the formation of skin-like structures, diverse techniques have been explored and used to fabricate scaffolds with nano- and microscale features to mimic the composition and structure of normal skin. Great progress has been made, and several of these products are commercially available to heal wounds as a temporary dressing or permanent substitutes, such as Alloderm® (LifeCell, Branchburg, NJ), Oasis® (Healthpoint, Fort Worth, TX), Integra® Dermal Regeneration Template (Integra Life Sciences, South Plainfield, NJ), and Biobrane® (Bertek Pharmaceuticals, Sugarland, TX). These scaffolds, in sponge, fibrous, or gel format, are produced from different polymers that support skin tissue formation. Salt leaching, emulsion freeze-drying, high-pressure gas expansion, phase separation, and electrospinning are common techniques to fabricate porous scaffolds for skin tissue formation [14]; these are further elaborated below. In addition, various parameters of biomimetic nanofibrous scaffolds that regulate tissue formation are discussed and their utilization for creating skin grafts is highlighted.
11.3.1 Particle Leaching Technique
The particle leaching method is one of the well-established techniques for fabricating porous scaffolds. This technique is based on a biodegradable polymer mixed with a salt in an organic solvent and then poured in a mold for evaporating the solvent. A structure with cellular pores can be obtained after the dissolution of the salt particle or porogen. Acceptable dimensional shrinkage may occur after salt leaching, but the structural integrity of scaffolds with microsized pores will be well maintained. By changing the size of salt particulates and the salt/polymer ratio, the pore size and porosity parameters of the scaffold can be controlled. Various porogens, such as salts, carbohydrates, and polymers can be used to produce porous materials. Water-soluble polymers of poly(ethylene oxide) (PEO) or gelatin can also be incorporated with some water-insoluble or slow-degrading polymers to produce artificial skin grafts [15]. Lee et al. [16] studied porous gelatin scaffolds that were prepared using a salt leaching method and compared them with scaffolds fabricated using a freeze-drying method for gelatin-containing artificial skin. The results showed that the scaffolds prepared from the salt leaching method had larger pore structures than that prepared by freeze-drying. After 1 week of in vitro culturing, fibroblasts showed a good affinity to the scaffold and were mainly distributed on the surface of the macropores. Sponges formed using the freeze-drying method had a dense surface that did not allow cells to penetrate into the inner areas. The mechanical strength and the rate of biodegradation were easily modulated by the addition of salt. It was found that the artificial dermis rather than the acellular sponge improved the reepithelialization on a full-thickness skin defect [16]. However, residual salts remaining in the scaffolds, rough morphologies of surface transferred from salt, irregularly shaped pores, and their poor interconnectivity pose challenges for cell seeding and culture [17].
11.3.2 Emulsion Freeze-Drying
Freeze-drying is one of the most extensively used methods to produce scaffolds with porosity greater than 90% [15]. The pore size depends on the growth rate of ice crystals during the freeze-drying process. In this method, an emulsion of a polymer with organic solvent and water is quickly frozen to set the pore structure before the two phases separate. Then the scaffold is freeze-dried to eliminate the residual solvents. After the removal of the frozen solvent, the remaining spaces become pores. This yields a fine porous scaffold compatible with cell cultures in tissue engineering. By adjusting the polymer concentration, using different solvents, or varying the cooling rate, phase separation could occur via different mechanisms, resulting in scaffolds with various morphologies. Although freeze-drying can prevent the disintegration of porous structure, it is so time- and energy-consuming that the whole scaffold fabrication process becomes inefficient and economically uncompetitive. Another problem encountered in the application of freeze-drying to the preparation of scaffolds is the occurrence of surface skin. During the freeze-drying stage, if the temperature is not kept sufficiently low, the polymer matrix is not rigid enough to resist the interfacial tension caused by the evaporation of the solvent. Freeze-dried porous scaffolds made from chitosan have been used to fabricate skin grafts; in particular, those collagen/chitosan composite scaffolds cross-linked by glutaraldehyde (GA) showed great potential as dermal equivalents with enhanced biostability and good biocompatibility [18].
11.3.3 High-Pressure Gas Expansion Methods
High-pressure gas expansion methods were developed to fabricate macroporous sponges from synthetic biodegradable polymers for potential use in tissue engineering without the use of organic solvents [19]. In this method, a biodegradable polymer was saturated with CO2 by exposure to high-pressure CO2 gas at room temperature in a pellet mold. Then the pressure was reduced to atmospheric levels rapidly to decrease the solubility of the gas in the polymer. This created a thermodynamic instability for the CO2 dissolved in the polymer disks, and resulted in the nucleation and growth of gas cells within the polymer matrix. By using this technique, open pores can be obtained within the polymer sponges. The porosity of the sponges could be controlled by the perform production technique, in which the disks preformed with compression molding or solvent casting were used to create pores with a certain size, then polymers such as polyglycolic acid (PGA) and poly(lactic-co-glycolic) acid (PLGA) were mixed in the disks to form foams with pore structure. Also, the porosity can be controlled by mixing crystalline and amorphous polymers. Fiber-reinforced foams could also be produced by placing polymer fibers within the polymer matrix before CO2 gas processing [20]. This matrix exhibits enhanced mechanical properties and can be utilized to form 3-D skin tissues. Also, this fabrication method may provide an ideal system for drug and/or growth factor incorporation into polymers used as skin tissue engineering matrices.
11.3.4 Phase Separation Method
The phase separation technique is based on thermodynamic demixing of a homogeneous polymer–solvent solution into a polymer-rich phase and a polymer-poor phase, usually by either exposure of the solution to another immiscible solvent or cooling the solution below a binodal solubility curve [21]. This cooling, known as thermally induced phase separation (TIPS), uses thermal energy as a latent solvent to induce phase separation [15]. The quenched polymer solution below the freezing point of the solvent is subsequently freeze-dried to produce porous structure. One of the advantages of this technique is that various porous structures can be easily obtained by adjusting various thermodynamic and kinetic parameters. The TIPS technique has been used commercially to produce microporous membranes for filtration, but the pore size of the resultant membrane is too small to be applied for cell seeding, which requires a pore diameter at least above 100 μM, with an open cellular morphology. The TIPS technique has been previously reported for the preparation of porous polylactic acid (PLA) scaffolds intended to use as tissue scaffolds. Although a wide array of microporous (1–10 μm) isotropic morphologies were reported in response to a slight change in the TIPS parameters, whether they have macroporous and open cellular structure throughout the matrix was not discussed [21]. Large pore size and an open porous structure are critical parameters for cell seeding and neovascularization when implanted in vivo. When compared with the prefabricated solid form, which requires additional and complicated processing, the solution-based phase separation technique is relatively simple and easy to manipulate. However, there have been limited studies on the selection of the porogen phase that can be properly available for the processing of biopolymer scaffolds.
11.3.5 Electrospinning Technique
Compared with the approaches mentioned earlier, electrospinning has recently received a tremendous amount of attention due to its low setup cost, easy operation, high production rate, good reproducibility, and the capability to fabricate fiber scaffolds from versatile materials. In addition, the nanofiber scaffolds have a large surface area to interact with cells and varying porosities and interfiber pore sizes controlling cellular infiltration. The electrospinning device contains a spinneret, a syringe pump, a high voltage supply, and a grounded conductive surface for fiber collection. Nanofibers fabricated by electrospinning closely mimic the architecture of native skin tissue at the nanometer scale. These nanofibrous matrices are ideal for skin tissue engineering applications due to their large surface area and distinct properties such as low density, high porosity, controllable pore size, and exceptional mechanical properties [22,23]. There are several parameters that can affect the electrospinning process and determine the physical properties of the obtained nanofibrous matrices [24–27]; these include polymer molecular weight, polymer solution properties, voltage, flow rate, distance between spinneret and collector (working distance), motion of the grounded collector, and ambient conditions (temperature, humidity, and air velocity). A variety of materials including synthetic and natural polymers (e.g., PLGA, poly-L-lactide (PLLA), polycaprolactone (PCL), poly(ethylene oxide terephthalate) (PEOT)-poly(butylene terephthalate) (PBT), collagen, chitosan, or blends of these materials) [28–33] have been successfully electrospun into micro- or nanosized fibers with diameters ranging from 50 to 1000 nm or greater [34,35]. Several synthetic and natural polymers that are used in electrospinning nanofibers for skin grafts have been briefly summarized in Table 11.1.
Table 11.1 Synthetic and Natural Polymers for Electrospun Nanofibers for Skin Grafts

The surfaces of most electrospun fibers are smooth with a solid cross-section. Efforts have been made to create secondary unique structures in the electrospun fibers, for example, core/shell composite fibers [36,37], tubular fibers [38], multichannel tubular structure [39], and porous fibers [40] to achieve novel properties and specific functionalities, such as the incorporation of drug molecules for controlled release [41] and promotion of cell anchorage [42] to the surface. Many potential applications can be identified with these structures. To fabricate either tubular or core/shell composite fibers, the coaxial electrospinning technique has been developed. By using a spinneret consisting of two coaxial capillaries with different diameters, two solutions can be accommodated for coelectrospinning. Inspired by this setup, a spinneret with multiple capillaries embedded in a plastic syringe at three vertices of an equilateral triangle was fabricated for multifluidic compound jet electrospinning [43].
The electrospinning technique also offers the opportunity to control the thickness and composition of the nanofibrous matrices [34,44–46]. Extensive efforts have been made to explore the potential utilization of electrospun nanofibers for tissue regeneration. Electrospun nanofiber scaffolds showed great advantages in designing biomimetic skin graft as a result of their morphological and dimensional similarity to the native skin ECM fibers [47] and facilitate the incorporation of various biomolecules including growth factors [48,49]. It has been demonstrated in a number of studies that nanofibrous scaffolds can promote cell growth and maintain proper phenotype [10–13]. All these unique properties of nanofibers have led to the effort to directly apply nanofibers for wound repair as a wound dressing/matrix or as scaffolds for skin tissue engineering applications [50–52]. There are several critical parameters that can influence skin tissue formation when using the electrospun nanofibrous matrices as skin grafts.
11.3.5.1 Pore Size
Interpore size, the size of the pores that are created by nanofibers, is one of the parameters that can influence skin tissue formation. The control of interfiber pore size is a critical issue especially in the case of cell infiltration and tissue ingrowth. The relationship between pore size and cell migration has been investigated and several results indicated that the cells could infiltrate into electrospun nanofiber mats with the average pore size of only 1.5 μm, which is much smaller than the minimum pore size of 10 μm assumed for cell infiltration in other scaffolds [53]. A possible explanation for this finding may result from the ability of cells to push aside individual fibers in the electrospun nonwoven mats. Lowery et al. [54] found that cells in the fibrous scaffolds with pores larger than 300 μm are no longer able to effectively bridge the interfiber gaps. Conversely, for those scaffolds with the pore sizes less than 1 μm, cell infiltration can be completely prevented (cell barrier) [54]. Based on these findings, an optimal pore size of 6–20 μm has been proposed for effective cell infiltration, which corresponds to approximately the average diameter of suspended cells. However, for most electrospun nanofiber meshes, the pore size is less than 5 μm, smaller than the cell size, which constrains the cells penetrating into the meshes [55]. A large number of studies have also looked into the effect of porosity and pore size on cell proliferation and infiltration by varying the fiber diameter [56–68]. However, the alteration of fiber diameter may lead to various unintended cellular responses, for example, cell proliferation and differentiation [54]. Obviously, a key challenge to systematically investigate the correlation between pore size and cell infiltration and cell proliferation is how to precisely control the pore size independent of other structural parameters, such as fiber diameter and porosity. With current electrospinning setups, this is very difficult to achieve especially considering the interdependency among fiber diameter, pore size, and porosity.
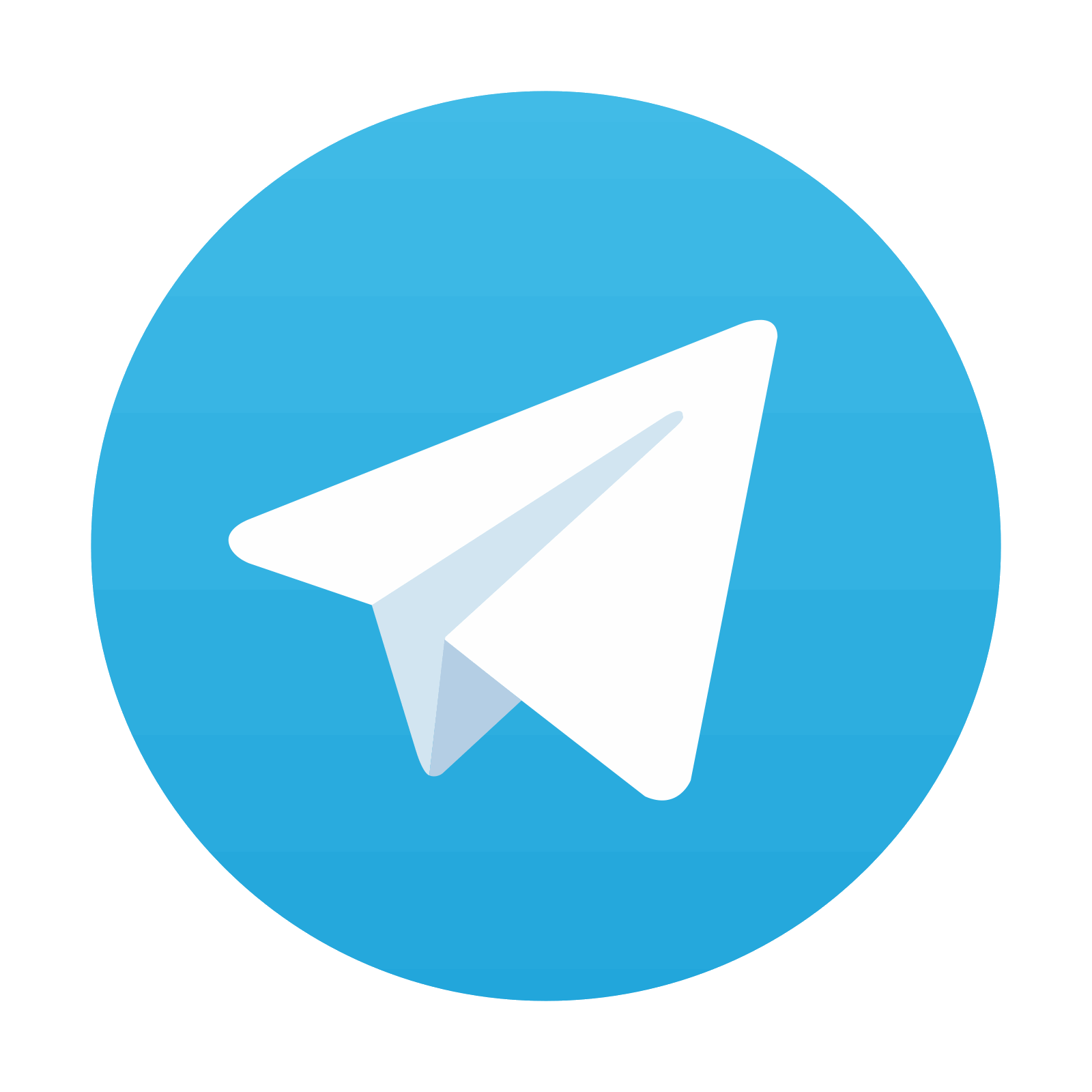
Stay updated, free articles. Join our Telegram channel
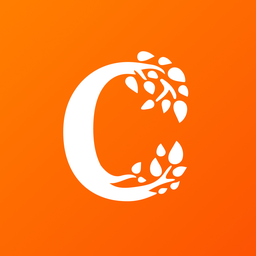
Full access? Get Clinical Tree
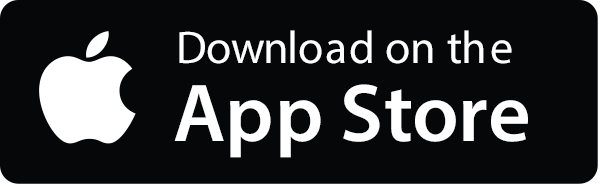
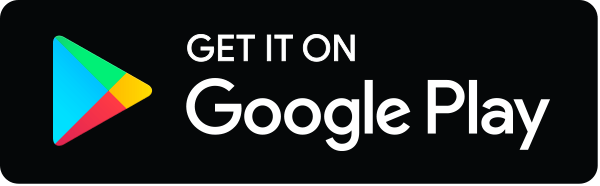