Epithelial Engineering: From Sheets to Branched Tubes
Departments of Chemical and Biological Engineering and Molecular Biology, Princeton University, Princeton, NJ, USA
10.1 Introduction
The epithelium is one of the major tissue types in the body, commonly arranged as either a single layer or multiple layers of cells [1,2]. Epithelial cells are polarized within the epithelium such that their apical surfaces face fluid-filled spaces, such as the inside of the tube, while their basal surfaces are juxtaposed to the extracellular environment (Figure 10.1A). Cohesion within this polarized epithelium is mediated by intercellular junctions, including tight junctions and desmosomes, thus enabling the tissue to form a contiguous sheet, which can fold and form a tubular structure. Notably, epithelia cover the surfaces of the body and line hollow tissues where they function to protect or enclose organs.
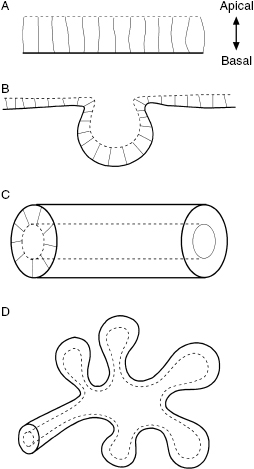
Construction of the basic structure and function of the epithelium begins during development, when populations of epithelial cells undergo dramatic changes in shape, including collective migration, bending, folding, or assembling a tube, which subsequently extends or branches to build a mature organ (Figure 10.1B–D). Here, we introduce examples of epithelial morphogenesis that occur in nature which can provide useful insights into how epithelial tissues sculpt themselves into various shapes. These morphogenetic behaviors illuminate gaps in our knowledge of regulatory cues, including the biochemical and mechanical signals that are present within the substratum, the soluble factors that direct cellular behaviors, and the intracellular machinery that drives epithelial shape changes. We also highlight engineering strategies that can be used to dissect the effects of the individual regulatory components by mimicking external environments and various stimuli during epithelial sheet migration, folding, tubulogenesis, and branching.
10.2 Inspiration from the Biology of Epithelial Morphogenesis
10.2.1 Collective Migration of Epithelial Sheets
The epithelium moves collectively to cover the whole embryo during the early stages of development, forms the internal lining of organs at later stages, and even fills holes during wound healing in the mature organism. These concerted epithelial movements are observed in many developmental processes, and tracking labeled cells within a moving epithelial sheet has provided significant insight into collective cell behaviors. For example, the animal–vegetal axis is the first one to form in the vertebrate embryo, with an animal pole that represents the future ectoderm and mesoderm and a vegetal pole that represents the future endoderm. The simple epithelium of the enveloping layer (EVL) of the zebrafish embryo moves toward the vegetal pole from the animal side, and eventually covers the entire embryo during a process known as epiboly (Figure 10.2A) [3]. These animal-to-vegetal movements of the epithelium are guided by underlying microtubule arrays that extend in parallel to the direction of migration [4], which is facilitated by pulling through contractions of the actin cytoskeleton and cell shape changes at the vegetal margin of the tissue (Figure 10.2A(d–f)) [5,6].
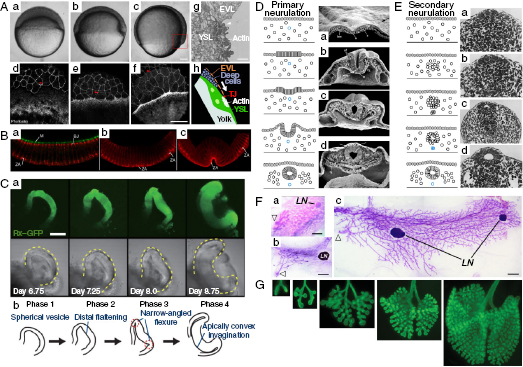
Epithelial sheets are usually accompanied by an underlying mesenchyme and extracellular matrix (ECM). Evidence of increased fibronectin assembly under the migrating epithelium [7] suggests a possible role for the ECM to act as a directional cue, which has been shown in some model systems [8,9]. Whereas the ECM has been thought to provide a guidance cue for migrating cells, live imaging has revealed that the ECM in the embryo is not fixed in place. Instead, analysis of the movements of ECM and epithelial cells suggest concerted motions [10], such that the cells move as flowing streams that merge at the primitive streak in avian embryos [11,12]. In the abdomen of Drosophila, the epidermal cells divide and then migrate in an oriented direction until the whole abdomen is covered [13], which differs from the oriented cell division that often controls epithelial morphogenesis. These examples suggest a major role for cell migration in embryonic development.
In addition to these morphogenetic movements, epithelial sheets migrate collectively to repair injured tissues. Unlike adult tissues, embryonic wounds do not induce an inflammatory response and heal rapidly without a scar [14]. Careful examination of the wound healing process in the epidermis of the wing bud of the embryonic chicken indicates that a contiguous F-actin cable, called the actin purse string, runs around the smooth margin of the wound and plays a major role in closing the wounded area [15]. In contrast, wound healing of the epithelium in Xenopus embryos requires the contraction of deep cells and epithelial protrusions, in addition to the actin purse string [16]. Epithelial cells can thus exhibit similar collective movements that are driven by disparate underlying physical mechanisms.
10.2.2 Folding of Epithelial Sheets
As epithelial tissues migrate to their final positions, these flat sheets begin to bend and fold to build additional dimensions into the embryo: by adding another germ layer during gastrulation, making hollow tubular structures during neurulation, or forming a spherical cup during eye development (Figure 10.2B–E). Tissue bending is an active process that has been proposed to use a number of mechanisms, including localized changes in cell shape driven by actomyosin contractions at the apical side of the epithelium, known as apical constriction (reviewed in Reference 17), differential cell proliferation, or differential positioning of adherens junctions [18]. These changes at localized regions of the mechanically linked sheet can cause it to bend. During gastrulation, apical constriction causes the epithelium to fold and, thereby, induces the tissue that covers the surface of the embryo to invaginate and penetrate inside to form an additional germ layer [19–22]. A similar mechanism of apical contractions drives gastrulation of the Drosophila embryo (Figure 10.2B) [23,24], where the dynamics of tissue folding has been best characterized for the formation of the ventral furrow, which internalizes to form a tube and marks the onset of gastrulation [25,26]. Live imaging and quantitative analysis of actin and myosin revealed that pulsed actomyosin contractions at the apical cortex pull the sites of adherens junctions inwards [26]. These contractions are stabilized incrementally between cycles through a ratchet-like mechanism, which constricts the apical side of a strip of ventral cells that fold into the embryo [26]. The polarized constrictions along the length of the cells of the ventral furrow result from the tissue-level tension integrated along the anterior–posterior axis [25].
In addition to this one-step folding of the epithelial sheet, further bending can arise at defined hinge points, where cell wedging takes place to provide additional nodes to fold. During primary neurulation in the mouse and chick (Figure 10.2D), the neural epithelium begins to form one acute bend (at the medial hinge point [MHP]) along the midline, resulting in a V-shaped groove, and later develops two additional dorsolateral hinge points (DLHPs), where subsequent inward bending occurs [27–30]. Additionally, the medial hinge cells have longer cell cycle times as compared with the others, which also enhances the folding of the neural epithelium [31].
More complex folding events have been uncovered during the morphogenesis of the optic cup using embryonic stem cell-derived neuroepithelial tissue (Figure 10.2C). Four distinct steps of morphogenesis were found to be controlled by local epithelial properties, driven by actomyosin contraction and subsequent tissue stiffening and tissue growth (Figure 10.2C) [32]. First, the monolayered epithelium bulges out from tissue aggregates with intense active myosin accumulation along the apical surface. Subsequently, during the second phase, the distal end flattens as the cells differentially contract, producing a flexible distal end and stiff lateral sides. During the third phase, cells in the lateral edge make a hinge point that causes inward buckling of the flattened distal epithelium. Invagination continues in a cell proliferation-dependent manner during the fourth and final phase [32]. Despite differences in underlying cellular mechanism, all epithelial folding results from these kinds of spatial patterns in cell behaviors.
10.2.3 Tubulogenesis
Epithelial tubes are essential functional units in many organs, acting as pipes that are used to transport the gases and liquids of the body. Epithelial tubes form with a distinct polarity in which the apical surface of the tissue faces the inside of a hollow lumen (Figure 10.1C). Several organs, including the neural tube, lung, kidney, and mammary gland, develop from simple epithelial tubes and their extensions, connections, and elaborations enable the construction of complex architectures. Tubular structures can be generated by two mechanisms: folding of the polarized epithelial sheet or by eliminating cells or producing a hollow lumen from an unpolarized cell mass. Neural tube formation is a well-known example, in which an epithelial tube is made by folding a polarized sheet via mechanisms described in the previous section. Once the epithelium folds, the lateral edges of the tissue must fuse together to form a closed tube (Figure 10.2D). To induce this open-ended epithelium to seal, the cells rearrange and change shape as they intercalate with each other in a direction perpendicular to the length of the tube under the control of the planar cell polarity (PCP) signaling pathway [33–36]; the elongated and actively moving cells cause the whole tissue to converge at the midline. In contrast, during secondary neurulation, the posterior neural tube forms from mesenchymal cells that condense into a solid rod and then transform into an epithelial tube (Figure 10.2E) [37,38]. Posterior neural tube formation in the avian embryo has especially distinguishable phases: formation of a medullary cord by dorsal cell aggregation, differentiation of central and peripheral cells, cavitation of multiple lumens, and coalescence of these into a single larger lumen [38]. A similar mechanism for tubulogenesis has been observed during formation of the gut in the zebrafish embryo [39].
In addition, tube formation during development of the salivary gland in Drosophila begins from a population of specified cells called the placode, which sequentially internalize through cell shape changes [40], rearrangement, and directed cell migration (for review, see References 41 and 42). Though assembly of the tubular structure is driven by various strategies, it requires dramatic epithelial cell movements and cell shape changes.
10.2.4 Branching Morphogenesis
The simple epithelial tubes described above often elongate, expand, and bifurcate at the ends to create a three-dimensional (3-D) tree-like structure via branching morphogenesis (Figure 10.1D), which generates a variety of organs including the mammary gland, salivary gland, kidney, and lungs. Each organ uses a unique process to build the complicated network of branched epithelial tubes [43]. Branching morphogenesis of the vertebrate submandibular gland (SMG) is especially well characterized. The spherical epithelial bud first forms shallow clefts along its surface that deepen and divide to generate multiple buds. The formation of these clefts is closely associated with fibronectin assembly [44–46], such that epithelial cells adjacent to fibronectin convert their cell–cell adhesions to cell–matrix adhesions as they communicate through a Rho kinase-mediated integrin and actomyosin network [47–49].
Unlike other branched organs, the mammary gland elaborates into a ramified structure during puberty in response to hormonal stimulation (Figure 10.2
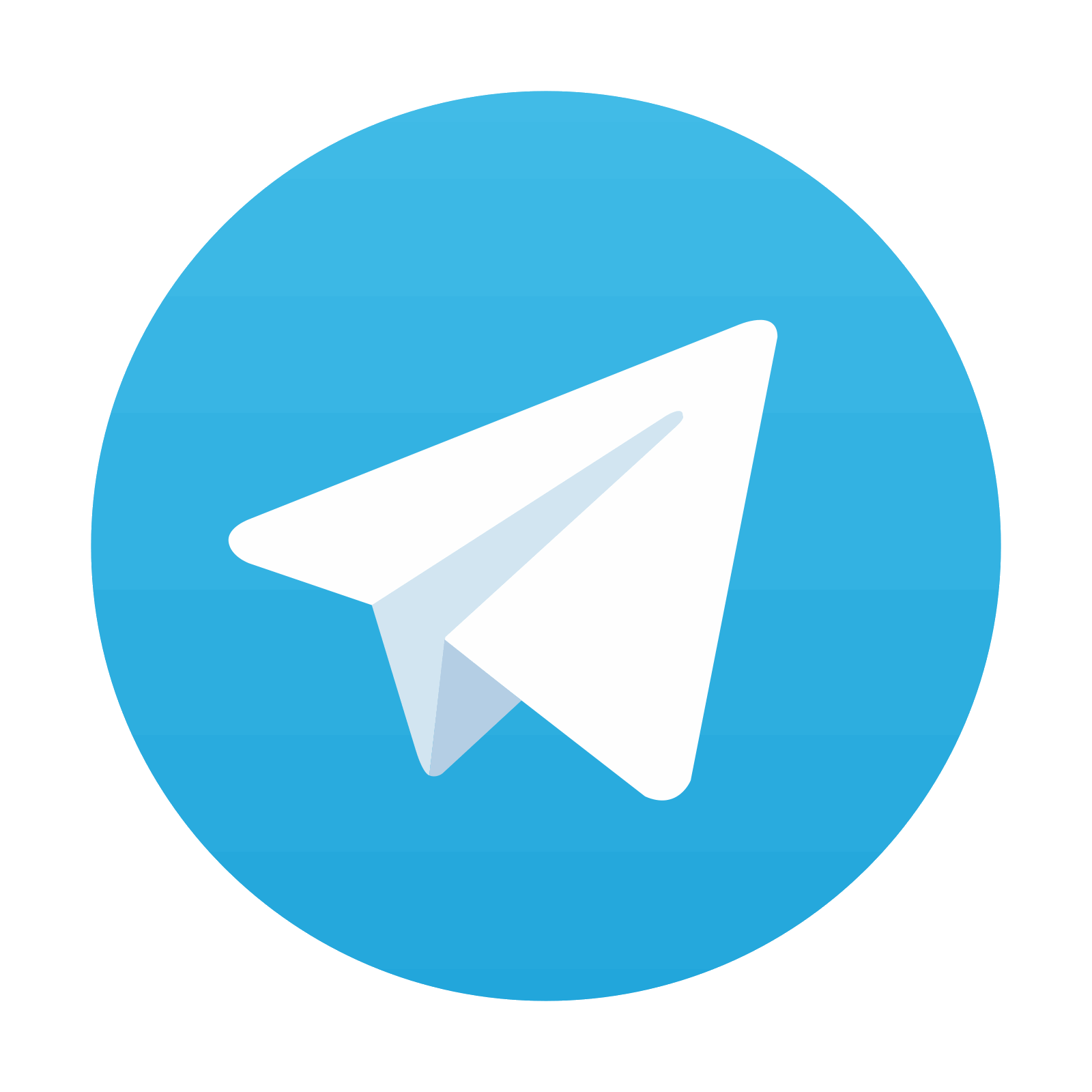
Stay updated, free articles. Join our Telegram channel
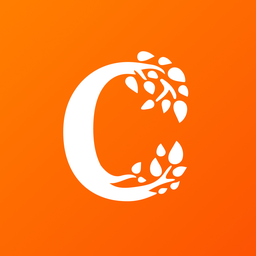
Full access? Get Clinical Tree
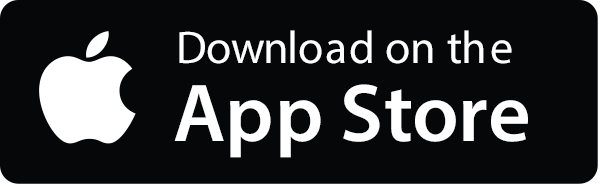
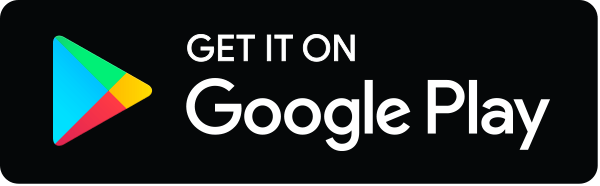