Introduction
Respiratory failure progressing to multiorgan failure is the primary cause of death in patients with COVID-19 infection. In most cases of COVID-19 disease, infection occurs when severe acute respiratory syndrome coronavirus 2 (SARS-CoV-2) enters the aerodigestive tract via aerosols or droplets.1 The major cell receptor necessary for the cellular entry of SARS-CoV-2 is the angiotensin-converting enzyme 2 (ACE2) in conjunction with cellular transmembrane protease serine 2 (TMPRSS2) found on the surface of epithelial cells lining the aerodigestive tract and the alveoli of the lungs.1,2 Initially, infection occurs in the nasopharynx and sinus epithelial cells and then in the bronchial tree and, in severe cases, in the distal airways and alveoli, resulting in bronchopneumonia.1,3–5 Although nasopharyngeal infection may result in anosmia, from a pathophysiologic standpoint, viral replication in the nasopharyngeal tract may be considered the asymptomatic phase, as the innate and adaptive immune system of the nasopharyngeal tract serves as the mainline of defense against progressive disease.1,5–7
Disease Progression
As the disease progresses into the distal lung, patients become symptomatic as a robust innate immune response occurs via the activation of interferon response genes and inflammasomes, followed by the release of interferons and proinflammatory cytokines.4,8,9 This host innate immune response results in viral clearance and resolution of the infection.4,10–12 However, SARS-CoV-2 encodes several proteins that allow the virus to evade host responses and blunt host interferon signaling.4,8,13,14 In approximately 20% of patients, perhaps due to impaired host viral recognition pathways, a blunted interferon response due to the possible formation of autoantibodies results in the virus not being cleared, followed by a pulmonary phase with the development of increasing pulmonary opacities and hypoxemia.15–19 During the pulmonary phase, viral infection of the gas exchange units of the lung consisting of type I and type II epithelial cells and endothelial cells is thought to occur. SARS-CoV-2 demonstrates a greater affinity to type II epithelial cells.20–23 Infection and inflammation results in a dysregulated immune response increasing type II epithelial cell apoptosis, pyroptosis, severe endothelial damage, and endothelial dysfunction.20–23 Increased activation and dysregulation of the innate immune response occurs due to type II epithelial apoptosis and pyroptosis with the increasing release of viable and nonviable viral particles and viral RNAemia into the alveolar unit.20–22,24
Approximately 5% of severely ill patients develop increasing pulmonary infiltrates, progressive hypoxemia, hyperinflammation, and coagulopathy, and are commonly described as developing COVID-19-associated acute respiratory distress syndrome (CARDS).20,25–27 Although labeled as ARDS, this is a misnomer due to the fact that in COVID this diagnosis of ARDS is based solely on the acute presence of bilateral opacities and hypoxemia, yet the traditional pathognomonic feature of alveolar edema is absent, as evidenced by the widely described high compliance of the lung in the early phase.28–31
Epidemiology
Several epidemiological studies reveal that among those COVID-19 patients who progress to the pulmonary phase and develop increasing severity of respiratory failure, approximately 29 to 89% require invasive mechanical ventilation (MV).32–36 In patients who require MV, variable and high mortality rates are observed.32–34,36 The variability observed in these studies is multifactorial and includes the timing of MV, the determination of the need for MV, availability of intensive care unit (ICU) resources, and burden of patient comorbidities.32–34,36,37 In ICU patients in general and in patients requiring MV in particular, predictors of mortality include age, lymphopenia, the burden of comorbidities, obesity, male gender, the persistent presence of viral RNAemia, and the severity of inflammation.20,24,38–41 The association between the presence of SARS-CoV-2 RNAemia and clinical outcome is controversial, with some studies demonstrating the association of RNAemia with respiratory failure and progressive organ dysfunction, and others demonstrating no association.24,42 Bermejo-Martin et al and others have demonstrated that higher levels of RNAemia are associated with the development of respiratory failure, multiorgan dysfunction, and coagulopathy.24 Schlesinger et al demonstrated that RNAemia was found in tracheal aspirates of critically ill patients with respiratory failure but there was no association with patient mortality.42 Although RNAemia may be accounted by increased viral load, it is most likely that the effects seen are most likely related to host response, immune dysregulation, and cytokine storm–associated inflammation.8,16,43,44
CARDS—Atypical ARDS or ARDS? Understanding Possible Variability
From the beginning of the pandemic, the observation was made that in many patients with severe COVID-19 pneumonia and associated respiratory failure (CARDS), there was a significant disconnect between the severity of hypoxemia, laboratory values, and the clinical presentation of the patient.45,46 Many patients were profoundly hypoxemic while having very little dyspnea (happy hypoxemics).45,47–49 However, the exact mechanism of asymptomatic hypoxemia has yet to be defined. A major potential cause is thought to involve pulmonary platelet activation and release of excess serotonin, which then causes abnormal hyperperfusion to the involved lung due to the lack of hypoxic vasoconstriction (serotonin causes vasodilation).47,50–53 Other factors include the development of distal fibroblast plugs consistent with organizing pneumonia (OP), possible viral effects on carotid body chemoreceptors and microvascular thrombosis.47,50–53 Many of these patients demonstrate preserved lung mechanics, including normal lung compliance. Rapid deterioration of clinical status has been observed in these patients.47,50–53
The major pulmonary pathologic findings in postmortem studies are, unsurprisingly, diffuse alveolar damage, given that this is the end result of all forms of lung injury, and a surprisingly high incidence of OP or acute fibrinous organizing pneumonia (AFOP) (Fig. 7.1a–d).52,54,55 A critical limitation of the pathologic literature is the near-complete lack of tissue biopsy in the early pulmonary phase. This is important because the radiology literature strongly suggests that, in virtually all cases, OP is the initial form of lung injury observed, likely explaining the profound response to corticosteroids.52,56 Furthermore, in most autopsy studies, COVID-19 patients who died of respiratory failure in contrast to historical controls of patients expiring from other forms of ARDS had a predominance of endotheliitis, microvascular injury, microthrombi, and thromboembolic disease.3,50,57 One study showed microvascular thrombosis affecting greater than 25% of the lung parenchyma in 87% of cases.3,50,57 Borczuk et al reported that although 71% of patients in their study received some form of anticoagulation, 46% demonstrated large vessel thrombosis, and 88% demonstrated microthrombosis in the pulmonary microcirculation.3
Fig. 7.1 (a) Normal alveoli. (https://www.newworldencyclopedia.org/entry/New_World_Encyclopedia:Creative_Commons_CC-by-sa_3.0; https://www.newworldencyclopedia.org/p/index.php?title=Pulmonary_alveolus&oldid=988660) (b) Diffuse alveolar damage (DAD). (https://www.newworldencyclopedia.org/p/index.php?title=Pulmonary_alveolus&oldid=988660; https://commons.wikimedia.org/wiki/User:Nephron/ https://fhs.mcmaster.ca/pathology/contact_us/faculty/faculty_bios/Bonert.html) (c) Diffuse alveolar damage with hyaline membranes (arrows). (Maiese, A., Manetti, A. C., La Russa, R., Di Paolo, M., Turillazzi, E., Frati, P., & Fineschi, V. (2021). Autopsy findings in COVID-19-related deaths: a literature review. Forensic science, medicine, and pathology, 17(2), 279–296. https://doi.org/10.1007/s12024-020-00310-8 http://creativecommons.org/licenses/by/4.0) (d) DAD with acute fibrinous and organizing pneumonia. (Gomes, R., Padrão, E., Dabó, H., Soares Pires, F., Mota, P., Melo, N., Jesus, J. M., Cunha, R., Guimarães, S., Souto Moura, C., & Morais, A. (2016). Acute fibrinous and organizing pneumonia: A report of 13 cases in a tertiary university hospital. Medicine, 95(27), e4073. http://creativecommons.org/licenses/by/4.0)
It is essential to note that during the SARS-CoV-1 epidemic OP and AFOP were reported in 30 to 60% of ICU cases, and in many respects, the clinical presentation, chest computed tomography (CT) radiographic findings, and postmortem studies suggest that CARDS represents a viral triggered OP.52,58 COVID-19 chest CT findings reveal the archetypal form of OP as evidenced by a temporal progression of disease from peripheral ground-glass opacities (GGO) to progressive bilateral GGO, organizing consolidations, and dense consolidations (Fig. 7.2).56,59–61
The initial clinical presentation of CARDS can be quite heterogeneous. Patients can be profoundly hypoxemic with no evidence of distress, or severely hypoxemic with normocapnia, hypocapnia, or hypercapnia. The response to nitrous oxide, ventilator recruitment procedures, and prone ventilation can be variable.28,29,62–65
Given that CARDS is distinct from “traditional” ARDS in that it initially presents with a lack of alveolar edema in early phases, a central focus of debate has been on the relative importance of the need to adhere to strict ARDS net protocols.28,29,64 Some observations and studies support little or no difference in CARDS versus ARDS patients, emphasizing there should be no deviation from lung-protective strategies and alveolar recruitment techniques.30,34,64,66 In contrast to ARDS from other causes, Gattinoni and colleagues reporting on 150 CARDS patients from northern Italy identified two different phenotypes of patients with CARDS, termed L and H phenotypes. Differing from ARDS, CARDS L phenotype representing 50% of their series demonstrated normal compliance for the degree of PaO2/FiO2 (low elastance), normal or near-normal lung water (low lung water), normal or near-normal expired gas volumes, low V/Q (low V/Q ratio), and poor recruitability of alveoli to maneuvers such as PEEP (low recruitability).28,29,31 CARDS H phenotype representing approximately 20 to 30% of cases are not dissimilar to ARDS from other causes manifesting low compliance (high elastance), high lung water, high right to left shunt, and high recruitability. It has been proposed that type L phenotype will progress to type H phenotype due to the severity of infection, host factors, immune phenomena, and self-induced lung injury (SILI) due to rising negative intrathoracic pressures as patients become increasingly dyspneic.28,29,67,68
The implementation of positive pressure of noninvasive positive-pressure ventilation or MV may also precipitate ventilator-induced lung injury.67,68 Chiumello et al compared chest CT scans and physiologic variables in CARDS patients and two different ARDS populations, and found that CARDS patients demonstrated higher compliance and nearly double the end-expiratory gas volume, CARDS patients had lower PaO2/FiO2 ratios when matched with ARDS patients with the same compliance, and, in contrast to ARS patients in whom PaO2/FiO2 decreases linearly with decreases in compliance, there was no such relationship in patients with CARDS.31 The investigators noted that increasing PEEP from 5 to 15 mm Hg in PaO2/FiO2 matched ARDS patients’ improved dead space, improved PACO2 and respiratory mechanics, and remained unchanged or worsened in patients with CARDS, suggesting that CARDS patients are at risk for alveolar overdistension and barotrauma.31 Grasselli et al, in a study comparing CARDS patients to a previously collected dataset of patients with ARDS, discovered that compliance was 28% higher, and up to 6% of patients with CARDS had compliance greater than 95%.30 Similar to the Chiumello cohort of patients, as PaO2/FiO2 decreased, static compliance decreased in the ARDS group, while in the CARDS group, this relationship with PaO2/FiO2 was not observed.30,31
Thus, the major cause of hypoxemic respiratory failure in CARDS is V/Q mismatch, with the major driving force of hypoxemia likely being the dysregulated microcirculatory function as opposed to the alveolar compartment in typical ARDS.28,29,31,69,70 In addition to the theory that excess serotonin from platelet activation underlies the pulmonary vasodilation in affected areas, other causes may be due to disruption of the normal homeostatic mechanism mediated by the local renin–angiotensin system of the microvascular endothelium.53,71 Although this occurs when the SARS-CoV-2 virus invades cells of the alveolar-capillary units, a common misconception of the pulmonary phase is that the opacities result from viral invasion and are then described as “viral pneumonia.” This is yet another misnomer, given that in only 20% of autopsies can viral cytopathic changes be found.72
Thus, although viral invasion is not common, it should be noted that it disrupts the healthy state when it occurs. Homeostasis is maintained by a balance of vasodilatory and vasoconstrictor molecules. Membrane bound ACE2 catalyzes angiotensin 2 (A2), a vasoconstrictive, inflammatory prothrombotic peptide, into Ang 1-7, which has anti-inflammatory, antithrombotic, vasodilatory properties.71,73,74 ACE2 also catalyzes A1 into Ang 1-9, a powerful activator of the vasodilatory bradykinin system. The binding of SARS-CoV-2 to membrane bound (ACE2) on susceptible cells of alveolar-capillary unit results in downregulation of ACE2; ADAM17 proteolytically cleaves soluble ACE2, resulting in further downregulation of ACE2. The result is a vasoplegic, proinflammatory, and prothrombotic state inducing microvascular thrombosis and loss of appropriate hypoxia-induced vasoconstriction.71,73,74
From the beginning of the pandemic, there has been a paradigm shift from early intubation in CARDS patients to a more stepwise approach favoring late intubation when necessary. Initially, due to fears of a rapid and sometimes unpredictable deterioration of CARDS patients, experts recommended early MV in patients who demonstrated severe hypoxemia that did not improve within 2 hours of increasing oxygen or other forms of noninvasive ventilation (NIV).75,76 Another concern was the development of SILI as a result of rapid swings in intrathoracic pressures due to increasing dyspnea and respiratory drive of spontaneously breathing hypoxemic CARDS patients receiving oxygenation with high-flow nasal cannula (HFNC) and other forms of NIV.28,67,68 Gattinoni et al, in a small series of patients, observed that patients transitioned from an L phenotype to an H phenotype after 1 week on NIV, suggesting that SILI was a potential initiating event.28 Furthermore, data from the LUNG SAFE study demonstrated increased mortality in patients with ARDS on NIV, which generated spontaneous tidal volumes greater than 9 mL/kg.77 Thus, there is no clear-cut threshold as to when a patient with CARDS should be initiated on MV. A recent meta-analysis and systematic review involving 12 studies and over 8,000 patients found no difference between the timing of MV and patient morbidity or mortality.78 Given the known higher mortality of MV, it appears reasonable to delay initiation as long as tolerable. Thus, current evidence is mounting supporting a stepwise conservative approach alongside close clinical monitoring of patients’ respiratory status in the decision to initiate MV.68,76,79–83
Management
Details on treatment and management of hospitalized patients will be extensively covered in other chapters and will briefly be mentioned in this section. Hypoxemia should not be the major criterion for MV. Therefore, we recommend a stepwise approach to respiratory support and try to delay intubation if at all possible, accepting permissive hypoxemia keeping O2 saturations greater than 80% unless there is evidence of oxygen debt demonstrated by lactic acidosis or desaturations of central venous blood (Table 7.1).84–90 Other causes of worsening or refractory hypoxemia should be sought as secondary bacterial infections, and large vessel thromboembolic events are not uncommon.91–94 Initial employment of oxygen support should begin with the administration of O2 via nasal cannula 1 to 6 L/min and escalation to HFNC up to 60 to 80 L/min if oxygenation goals are not met.86,87,95 One should consider MV in patients on NIV who generate tidal volumes of greater than 9 mL/kg despite measures to attenuate large swings in intrathoracic pressures.77 If tolerated, awake prone positioning should be attempted. Should patients require MV, volume-protective strategies should be employed with the lowest driving pressure (<15 cm H2O) and lowest possible PEEP. If the decision to employ prone MV is made, it should be maintained for 16 to 18 hours.86,87,95 Neuromuscular blockade and sedation may be needed (Table 7.1).
COVID-19 respiratory disease, best described as secondary OP in its early stages and CARDS later, is a severe inflammatory disease highly responsive to glucocorticoid therapy.96–100 The benefits of therapy appear to be time-dependent.97–99,101 Early aggressive treatment is critical to prevent disease progression, as delays in therapy may limit disease reversibility. Thus, the first task of the clinician is to determine the reversibility of the pulmonary disease by assessing the time of onset of symptoms and employing chest CT to determine the extent and stage of pulmonary involvement as GGO pattern is significantly more prevalent in early-phase disease compared with the late-phase disease. In contrast, consolidation patterns are significantly more common in the late phase.61,102–105 Monitoring D-dimer and C-reactive protein (CRP) may assist in predicting outcome.20,106 Intubated patients who have low pulmonary compliance and elevated D-dimers or progressive elevations in D-dimer are associated with poor prognosis.30 Elevated CRP levels with declines in levels upon initiation of anti-inflammatory/immunomodulatory therapy suggest reversibility of pulmonary disease.97,105,107,108
In patients requiring respiratory support, glucocorticoid therapy has been shown to reduce mortality.100 The RECOVERY trial randomized 2,104 patients to receive dexamethasone 6 mg (equivalent to 32-mg methylprednisolone) once daily (by mouth or intravenous injection) for 10 days, versus 4,321 patients who received standard treatment.100 In ventilated patients, dexamethasone reduced mortality by roughly 30% (rate ratio, 0.65 [95% confidence interval, 0.48– 0.88]; p = 0.01).100 A recent study from the COVID-19 SPANISH ICU Network demonstrated that pre-ICU corticosteroids and corticosteroids administered within 48 hours of admission to the ICU reduced mortality.101 However, patients who received late corticosteroids had an increase in mortality and a high risk for secondary infection.101 Their study underscores the importance of early anti-inflammatory therapy in the pulmonary phase of COVID-19. Furthermore (and most importantly), early high-dose corticosteroids, far higher than those employed in the RECOVERY trial of dexamethasone (>1 mg/kg methylprednisolone eq/day), were associated with far more reduced mortality compared to early low-dose corticosteroids.101
Studies employing methylprednisolone and hydrocortisone in CARDS are described in Table 7.2.97,98,109–114
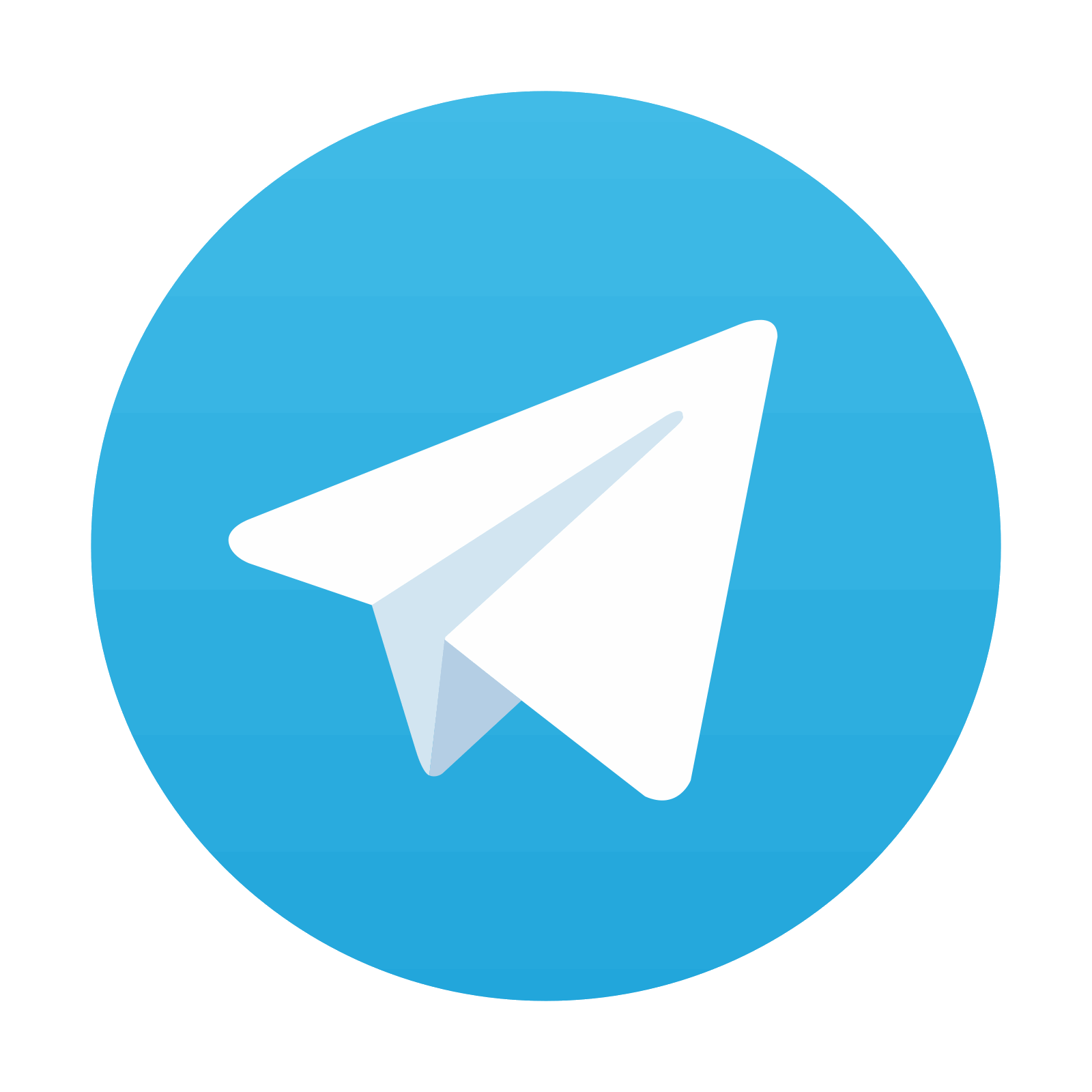
Stay updated, free articles. Join our Telegram channel
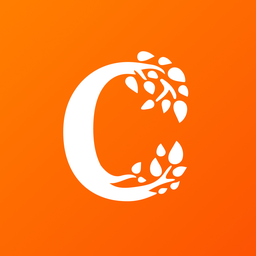
Full access? Get Clinical Tree
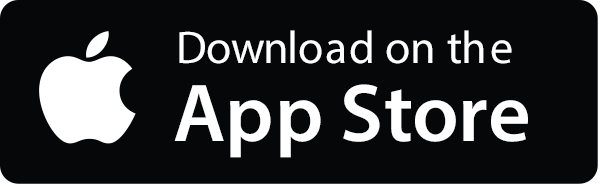
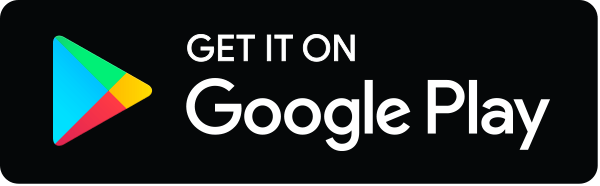
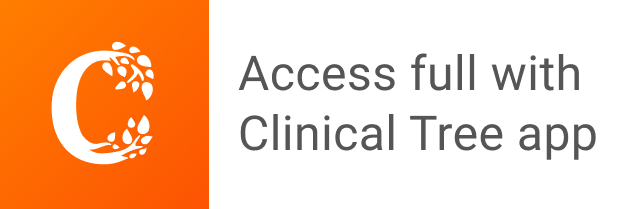