Chapter 15 Plasma Proteins
Plasma contains approximately 0.9% inorganic ions, 0.8% small organic molecules (more than half of this is lipid), and 7% protein (Table 15.1). A pink coloration of the plasma suggests hemolysis, either in the patient or, more often, in the test tube as a result of careless handling. A milky appearance, or the formation of a fatty layer during centrifugation, shows the presence of chylomicrons, which are small fat droplets that appear in the plasma after a fatty meal. A turbid appearance in the fasting state suggests a hypertriglyceridemia with elevated very-low-density lipoprotein.
Table 15.1 Reference Values for Some Plasma Constituents
Plasma Constituent | Reference Value |
---|---|
Gases and Electrolytes | |
pO2 arterial | 95–100 mmHg |
CO2 arterial | 21–28 mmol/L |
CO2 venous | 24–30 mmol/L |
HCO3− | 21–28 mmol/L |
Cl− | 95–103 mmol/L |
Na+ | 136–142 mmol/L |
K+ | 3.8–5.0 mmol/L |
Ca2+ (total) | 2.3–2.74 mmol/L |
Mg2+ | 0.65–1.23 mmol/L |
pH | 7.35–7.44 |
Metabolites | |
Glucose (fasting) | 3.9–6.1 mmol/L (70–110 mg/dl) |
Ammonia | 7–70 μmol/L (12–120 μg/dl) |
Urea nitrogen | 2.9–8.2 mmol/L (8–23 mg/dl) |
Uric acid | 0.16–0.51 mmol/L (2.7–8.5 mg/dl) |
Creatinine | 53–106 μmol/L (0.6–1.2 mg/dl) |
Bilirubin (total) | 2–20 μmol/L (0.1–1.2 mg/dl) |
Bile acids | 0.3–3 mg/dl |
Lipids (total) | 400–800 mg/dl |
Acetoacetic acid | 20–100 μmol/L (0.2–1 mg/dl) |
Acetone | 50–340 μmol/L (0.3–2 mg/dl) |
Proteins | |
Total protein | 6–8 g/dl |
Albumin | 3.2–5.6 g/dl (52%–65% of total) |
α1-Globulins | 0.1–0.4 g/dl (2.5%–5% of total) |
α2-Globulins | 0.4–1.2 g/dl (7%–13% of total) |
β-Globulins | 0.5–1.1 g/dl (8%–14% of total) |
γ-Globulins | 0.5–1.6 g/dl (12%–22% of total) |
The blood pH is tightly regulated
Carbonic acid/bicarbonate is the most important physiological buffer system in the body. It is important because CO2 and HCO3− are present in high concentrations in the interstitial and intracellular compartments as well as the plasma (Fig. 15.1). In addition, the CO2 level can be regulated by the lungs and the HCO3− level by the kidneys.
Phosphate groups provide an additional buffer system:
Acidosis and alkalosis are common in clinical practice
Respiratory acidosis is caused by the abnormal retention of CO2, and respiratory alkalosis is caused by hyperventilation. For example, a doubling in the rate of alveolar ventilation raises the blood pH from 7.40 to 7.62, and a 50% reduction in alveolar ventilation lowers the blood pH from 7.40 to 7.12 (Fig. 15.2).
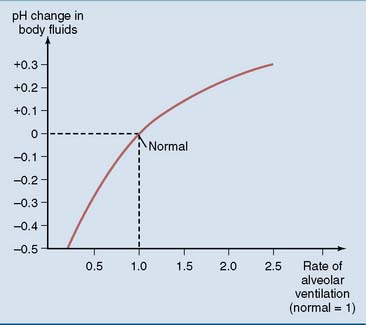
Figure 15.2 pH change in plasma and extracellular fluids in response to changes in alveolar ventilation.
Plasma proteins are both synthesized and destroyed in the liver
With the important exception of albumin, most plasma proteins are glycoproteins. Their N-linked oligosaccharides end with sialic acid (N-acetylneuraminic acid) bound to galactose. A typical plasma protein remains in the circulation for several days, and during this time the terminal sialic acid residues are gradually chewed off by endothelial neuraminidases (Fig. 15.3). After the loss of the sialic acid, the exposed galactose at the end of the oligosaccharide binds to an asialoglycoprotein receptor on the surface of hepatocytes, followed by receptor-mediated endocytosis and lysosomal degradation.
Albumin prevents edema
Electrophoresis is the most important method for the separation of plasma proteins in the clinical laboratory (Fig. 15.4). It usually is performed at alkaline pH on a solid or semisolid support such as cellulose acetate foil or an agarose gel. This method separates the proteins by their charge/mass ratio. Five fractions can be identified by staining and densitometric scanning: albumin, and the α1-, α2-, β-, and γ-globulins.
The colloid osmotic pressure is necessary to prevent edema. The hydrostatic pressure of the blood forces fluid from the capillaries into the interstitial spaces, and the colloid osmotic pressure of the plasma proteins is required to pull the fluid back into the capillaries (Fig. 15.5).
Albumin binds many small molecules
The dissociation constant KD for the release of the drug from albumin is defined as
As long as the molar concentration of albumin is far higher than that of the drug, the concentration of free, unbound albumin [Alb] approximates the total serum albumin concentration. Equation (2) indicates that in a patient whose albumin concentration is only half of normal, the ratio of free drug/bound drug is doubled. This is important because only the fraction of the drug that is not protein bound in the plasma equilibrates freely with the tissues. Therefore it is not the total drug concentration, but the concentration of the unbound drug, that determines the biological response.
Some plasma proteins are specialized carriers of small molecules
Many of the proteins listed in Table 15.2 are specialized binding proteins that ferry endogenous substances through the blood.
Haptoglobin and hemopexin are binding proteins with a very different function. After intravascular hemolysis, the hemoglobin that is released from the ruptured erythrocytes dissociates into α-β dimers that are too small (MW 33,000D) to escape renal excretion. To prevent the loss of hemoglobin with its valuable iron, the hemoglobin binds to haptoglobin, and any free heme binds to hemopexin. These complexes are cleared by phagocytic cells and hepatocytes, respectively (Fig. 15.6).
Levels of plasma proteins are affected by many diseases
Plasma protein electrophoresis is a valuable aid in the diagnosis of many diseases. Figure 15.7 summarizes some typical patterns.
Acute-phase reactants are plasma proteins whose levels change within 1 or 2 days after acute trauma or surgery, and especially during infections and inflammation (Table 15.3). Their synthesis is controlled by stress hormones and cytokines that are released in these conditions. The albumin peak is reduced, whereas the α2 peak is often increased because one of its major components, haptoglobin, is a positive acute-phase reactant.
Table 15.3 Acute-Phase Reactants*
Protein | Fraction | Response |
---|---|---|
Albumin | Albumin | ↓ |
α1-Acid glycoprotein | α1 | ↑↑ |
α1-Antiprotease | α1 | ↑ |
Ceruloplasmin | α2 | (↑) |
Haptoglobin | α2 | ↑↑ |
α2-Macroglobulin | α2 | ↓ |
Fibrinogen | β/γ | ↑ |
C-reactive protein | β/γ | ↑↑↑ |
* The levels of these plasma proteins are either elevated or reduced in many acute illnesses.
Abnormalities in the concentrations of minor plasma proteins cannot be divined from the electrophoretic pattern and have to be determined by sensitive immunological methods. For example, α-fetoprotein is synthesized in the fetal liver but occurs in only trace amounts in normal adult blood. Its levels are increased in most patients with hepatocellular carcinoma. Effectively, the cancer cells revert to a fetal phenotype that entails α-fetoprotein production in addition to rapid proliferation. More importantly, α-fetoprotein is used for the prenatal diagnosis of open neural tube defects. In these severe malformations, α-fetoprotein leaks from the fetal blood into amniotic fluid and even into the maternal blood (Fig. 15.8).
Blood components are used for transfusions
Blood transfusions necessitate blood group matching, and there is a risk of transmitting acquired immunodeficiency syndrome (AIDS), hepatitis, and other diseases. Also, not every patient requires the same blood component. An anemic patient needs red blood cells, a patient with nephrotic syndrome needs albumin, a patient with a clotting disorder needs clotting factors or platelets, and a patient with an immunodeficiency can be treated with immunoglobulins. Table 15.4 lists some of the most important blood products and their uses.
Table 15.4 Plasma Components Available for Therapeutic Use
Product | Uses | Comments |
---|---|---|
Fresh-frozen plasma | Multiple clotting factor deficiencies, liver cirrhosis, disseminated intravascular coagulation | Danger of disease transmission |
Cryoprecipitate | Clotting disorders: hypofibrinogenemia, hemophilia, von Willebrand disease | Produced by freezing and thawing of plasma; enriched in fibrinogen, factor VIII, fibronectin |
Factor VIII concentrate | Hemophilia A | Some danger of hepatitis transmission |
Albumin 5% | Hypovolemic shock | No danger of hepatitis or HIV transmission; no blood group antibodies present |
Albumin 25% | Cerebral edema | |
Immune serum globulin | Immunodeficiency states affecting B cells; passive immunization against hepatitis, tetanus | For intravenous or intramuscular injection |
Immunoglobulins bind antigens very selectively
Antibodies consist of two light chains and two heavy chains
All antibodies have the same general structure, as shown for immunoglobulins of the G1 class (IgG1) in Figure 15.9. The molecule consists of four disulfide-bonded polypeptides: two identical heavy chains with MW of 53,000 D each and two identical light chains with MW of 23,000 D each. The molecule has the shape of the letter Y. Each of the two arms of the Y is formed by the amino-terminal half of a heavy chain and a complete light chain. The stem consists of the carboxyl-terminal halves of the two heavy chains.