KEY POINTS
Thrombolytic therapy, surgical thrombectomy, and placement of inferior vena cava filters are adjunctive treatments that may be indicated in patients with extensive and complicated venous thromboembolism.
Deep vein thrombosis (DVT) and pulmonary embolism are frequent complications after major abdominal and orthopedic procedures. The risk is further increased in patients with malignancy and a history of venous thromboembolism. Options for DVT prophylaxis include intermittent pneumatic compression, use of graduated compression stockings, and administration of low-dose unfractionated heparin, low molecular weight heparin, fondaparinux, and vitamin K antagonists. However, prophylaxis should be stratified based on the patient’s level of risk.
In patients with established DVT, unfractionated heparin, low molecular weight heparin, and fondaparinux are options for initial antithrombotic therapy. The duration and type of long-term anticoagulation should be stratified based on the provoked or unprovoked nature of the DVT, the location of the DVT, previous occurrence of DVT, and presence of concomitant malignancy.
Saphenous vein stripping, endovenous laser treatment, and radiofrequency ablation are effective therapies for patients with saphenous vein valvular insufficiency. Concomitant varicose veins may be managed with compression therapy, sclerotherapy (for smaller varices), and phlebectomy.
The mainstay of treatment for chronic venous insufficiency is compression therapy. Sclerotherapy, perforator vein ligation, and venous reconstruction may be indicated in patients in whom conservative management fails.
Lymphedema is categorized as primary (with early or delayed onset) or secondary. The goals of treatment are to minimize edema and prevent infection. Lymphatic massage, sequential pneumatic compression, use of compression garments, and limb elevation are effective forms of therapy.
VENOUS ANATOMY
Veins are part of a dynamic and complex system that returns low-nutrient deoxygenated blood to the heart. Venous blood flow is dependent on multiple factors such as gravity, venous valves, the cardiac and respiratory cycles, blood volume, and the calf muscle pump. Alterations in the intricate balance of these factors can result in venous pathology.
Veins are thin-walled, highly distensible, and collapsible. Their structure specifically supports the primary functions of veins to transport blood toward the heart and serve as a reservoir to prevent intravascular volume overload.
The venous intima is composed of a nonthrombogenic endothelium with an underlying basement membrane and an elastic lamina. The endothelium produces endothelium-derived relaxing factors such as nitric oxide and prostacyclin, which help maintain a nonthrombogenic surface through inhibition of platelet aggregation and promotion of platelet disaggregation.1 Circumferential rings of elastic tissue and smooth muscle located in the media of the vein allow for changes in vein caliber with minimal changes in venous pressure. The adventitia is most prominent in large veins and consists of collagen, elastic fibers, and fibroblasts. When a vein is maximally distended, its diameter may be several times greater than that in the supine position.
In the axial veins, unidirectional blood flow is achieved with multiple venous valves. The inferior vena cava (IVC), common iliac veins, portal venous system, and cranial sinuses are valveless. In the axial veins, valves are more numerous distally in the extremities than proximally. Each valve consists of two thin cusps of a fine connective tissue skeleton covered by endothelium. Venous valves close in response to cephalad-to-caudal blood flow at a velocity of at least 30 cm/s.2
Lower extremity veins are divided into superficial, deep, and perforating veins. The superficial venous system lies above the uppermost fascial layer of the leg and thigh and consists of the great saphenous vein (GSV) and small saphenous vein (SSV) and their tributaries. The GSV originates from the dorsal pedal venous arch and courses cephalad, anterior to the medial malleolus, entering the common femoral vein approximately 4 cm inferior and lateral to the pubic tubercle. The saphenous nerve accompanies the GSV medially from the ankle to the level of the knee and supplies cutaneous sensation to the medial leg and ankle. The SSV originates laterally from the dorsal pedal venous arch and courses cephalad in the posterior calf. Most often, it penetrates the popliteal fossa, between the medial and lateral heads of the gastrocnemius muscle, to join the popliteal vein. The termination of the SSV may be quite variable, however, with a proximal extension of the SSV (the vein of Giacomini) frequently connecting with the deep femoral vein or GSV. The sural nerve accompanies the SSV laterally along its course and supplies cutaneous sensation to the lateral malleolar region.
The deep veins follow the course of major arteries in the extremities. In the lower leg, paired veins parallel the course of the anterior tibial, posterior tibial, and peroneal arteries, to join behind the knee forming the popliteal vein. Venous bridges connect the paired veins in the lower leg. The popliteal vein continues through the adductor hiatus to become the femoral vein. In the proximal thigh, the femoral vein joins with the deep femoral vein to form the common femoral vein, becoming the external iliac vein at the inguinal ligament.
Multiple perforator veins traverse the deep fascia to connect the superficial and deep venous systems. Potentially clinically important perforator veins are the Cockett and Boyd perforators. The Cockett perforator veins drain the medial lower leg and are relatively constant. They connect the posterior arch vein (a tributary to the GSV) and the posterior tibial vein. They may become varicose or incompetent in venous insufficiency states. The Boyd perforator veins connect the GSV to the deep veins approximately 10 cm below the knee and 1 to 2 cm medial to the tibia.
Venous sinuses are thin-walled, large veins located within the substance of the soleus and gastrocnemius muscles. These sinuses are valveless and are linked by valved, small venous channels that prevent reflux. A large amount of blood can be stored in the venous sinuses. With each contraction of the calf muscle bed, blood is pumped out through the venous channels into the main conduit veins to return to the heart.
As in the lower extremity, there are deep and superficial veins in the upper extremity. Deep veins of the upper extremity are paired and follow the named arteries in the arm. Superficial veins of the upper extremity are the cephalic and basilic veins and their tributaries. The cephalic vein originates at the lateral wrist and courses over the ventral surface of the forearm. In the upper arm, the cephalic vein terminates in the infraclavicular fossa, piercing the clavipectoral fascia to empty into the axillary vein. The basilic vein runs medially along the forearm and penetrates the deep fascia as it courses past the elbow in the upper arm. It then joins with the deep brachial veins to become the axillary vein. The median cubital vein joins the cephalic and the basilic veins on the ventral surface of the elbow.
The axillary vein becomes the subclavian vein at the lateral border of the first rib. At the medial border of the scalenus anterior muscle, the subclavian vein joins with the internal jugular vein to become the brachiocephalic vein, with the subclavian vein coursing anterior to the scalenus anterior muscle. The left and right brachiocephalic veins join to become the superior vena cava, which empties into the right atrium.
EVALUATION OF THE VENOUS SYSTEM
Evaluation of the venous system begins with a detailed history and physical examination. Risk factors for acute and chronic venous disease are identified. They include increased age, history of venous thromboembolism (VTE), malignancy, trauma and spinal cord injury, hospitalization and immobilization, obesity, nephrotic syndrome, pregnancy and the recently postpartum state, oral contraceptive use or hormone replacement therapy, varicose veins, and hypercoagulable states, as well as the postoperative state. Venous pathology is often, but not always, associated with visible or palpable signs that can be identified during the physical examination. There is variation among individuals in the prominence of superficial veins when the person is standing (Fig. 24-1). The superficial veins of a lean athletic person, even when normal, will appear large and easily visualized, but these veins will be far less obvious in the obese individual. Possible signs of superficial venous abnormalities are listed in Table 24-1. The deep veins cannot be directly assessed clinically, and abnormalities within them can only be inferred indirectly from changes found on clinical examination.
Chronic venous insufficiency (CVI) may lead to characteristic changes in the skin and subcutaneous tissues in the affected limb. CVI results from incompetence of venous valves, venous obstruction, or both. Most CVI involves venous reflux, and severe CVI often reflects a combination of reflux and venous obstruction. It is important to remember that although CVI originates with abnormalities of the veins, the target organ of CVI is the skin, and the underlying physiologic and biochemical mechanisms leading to the cutaneous abnormalities associated with CVI are poorly understood. A typical leg affected by CVI will be edematous, with edema increasing over the course of the day. The leg may also be indurated and pigmented with eczema and dermatitis. These changes are associated with excessive proteinaceous capillary exudate and deposition of a pericapillary fibrin cuff that may limit nutritional exchange. In addition, an increase in white blood cell trapping within the skin microcirculation in CVI patients may lead to microvascular congestion and thrombosis. Subsequently, white blood cells may migrate into the interstitium and release necrotizing lysosomal enzymes, potentially leading to tissue destruction and eventual ulceration.
Fibrosis can eventually develop from impaired nutrition, chronic inflammation, and fat necrosis (lipodermatosclerosis). Hemosiderin deposition due to the extravasation of red cells and subsequent lysis in the skin contributes to the characteristic pigmentation of chronic venous disease (Fig. 24-2). Ulceration can develop with longstanding venous hypertension and is associated with alterations in microcirculatory and cutaneous lymphatic anatomy and function. The most common location of venous ulceration is approximately 3 cm proximal to the medial malleolus (Fig. 24-3).
Trendelenburg’s test is a clinical test, historically important but now rarely used, that can help determine whether incompetent valves are present and in which of the three venous systems (superficial, deep, or perforator) the valves are abnormal. There are two components to this test. First, with the patient supine, the leg is elevated 45° to empty the veins, and the GSV is occluded with the examiner’s hand or with a rubber tourniquet. With the GSV still occluded, the patient stands and the superficial veins are observed for blood filling. The compression on the GSV is released and the superficial veins are observed for filling with blood. A negative result, indicating no clinically relevant venous reflux, is the gradual filling of the veins from arterial inflow. A positive result is the sudden filling of veins with standing while the GSV remains occluded indicating incompetent perforator and deep veins. The GSV valves are incompetent if the second component of the test yields a positive result. Interpretation of the findings of Trendelenburg’s test is subjective, and therefore, it has largely been supplanted by the more objective noninvasive vascular laboratory tests to localize sites of venous reflux.
Before the development of vascular ultrasound, noninvasive techniques to evaluate the venous system were based on plethysmographic techniques. Although a variety of plethysmographic techniques are used in the evaluation of both acute and chronic venous disease, they are all based on the detection of volume changes in the limb in response to blood flow.
Duplex ultrasonography (DUS) augmented by color flow imaging is now the most important noninvasive diagnostic method in the evaluation of the venous system. DUS has become standard for the detection of infrainguinal deep vein thrombosis (DVT), with near 100% sensitivity and specificity in symptomatic patients.3 It is also the preferred method of evaluation for upper extremity venous thrombosis and is useful in the evaluation of CVI by documenting the presence of valvular reflux and venous obstruction. Overlying bowel gas and large body habitus make DUS less applicable to evaluation of intra-abdominal veins. Magnetic resonance venography (MRV) and computed tomography (CT) venography are noninvasive techniques for evaluation of pelvic and intra-abdominal veins.
Improved accuracy of noninvasive techniques for diagnostic purposes has made the use of invasive procedures more selective. Both venography and intravascular ultrasound (IVUS) are used as adjuncts to percutaneous or open surgical treatment of venous disorders. When planning endovascular or open surgical treatment, venography may be used to identify areas of obstruction in infrainguinal, intra-abdominal, and upper extremity veins as well as reflux in intra-abdominal and infrainguinal veins. IVUS, with access generally via the common femoral vein, is used primarily to assess for occlusive lesions of the iliac veins and appears more sensitive than venography in detecting iliac vein obstruction.
Complications of venography include pain, thrombosis, or hematoma at the puncture site. Pain is lower with nonionic low-osmolality contrast media than with conventional contrast agents (with 18% vs. 44% of patients experiencing discomfort, respectively).4 Systemic effects of iodinated contrast media include allergic reaction and risk of renal failure. Postvenography venous thrombosis occurs distal to the venous puncture site in 1% to 9% of patients undergoing venography secondary to intimal damage from the intravenous (IV) contrast agent.4 Complications of IVUS are primarily related to the access site.
VENOUS THROMBOEMBOLISM
Despite increased awareness and use of prophylactic modalities, DVT and pulmonary embolism (PE), or VTE, remain important preventable sources of morbidity and mortality, especially in the surgical patient. The incidence of VTE is approximately 100 per 100,000 people per year in the general population, with 20% of the diagnoses made within 3 months of a surgical procedure. Of the symptomatic patients, one third will present with PE and two thirds with DVT.5,6 The estimated number of cases of VTE may well be over 600,000 per year in the United States, making it a major U.S. health problem.7 Furthermore, death occurs in 6% of DVT and 12% of PE cases within 1 month of diagnosis.5 Not only does VTE pose a veritable threat to life, but it also places patients at higher risk for recurrence and post-VTE sequelae such as pulmonary hypertension and postthrombotic syndrome, with 4% and up to 30% incidence, respectively.8,9,10
Three conditions, first described by Rudolf Virchow in 1862, contribute to VTE formation: stasis of blood flow, endothelial damage, and hypercoagulability. Of these risk factors, relative hypercoagulability appears most important in most cases of spontaneous VTE, or so-called idiopathic VTE, whereas stasis and endothelial damage likely play a greater role in secondary VTE, or so-called provoked VTE, occurring in association with transient risk factors such as immobilization, surgical procedures, and trauma. Identifiable risk factors for VTE generally relate to one of the conditions described by Virchow. Often more than one risk factor is present. Specific risk factors for VTE are listed in Table 24-2.
Acquired Advanced age Hospitalization/immobilization Hormone replacement therapy and oral contraceptive use Pregnancy and puerperium Prior venous thromboembolism Malignancy Major surgery Obesity Nephrotic syndrome Trauma or spinal cord injury Long-haul travel (>6 hours) Varicose veins Antiphospholipid antibody syndrome Myeloproliferative disease Polycythemia Inherited Factor V Leiden Prothrombin 20210A Antithrombin deficiency Protein C deficiency Protein S deficiency Factor XI elevation Dysfibrinogenemia Mixed Etiology Homocysteinemia Factor VII, VIII, IX, XI elevation Hyperfibrinogenemia Activated protein C resistance without factor V Leiden |
The more common acquired VTE risk factors include older age (>40 years), hospitalization and immobilization, hormone replacement and oral contraceptive therapy, pregnancy and the recently postpartum state, prior VTE, malignancy, major surgery, obesity, nephrotic syndrome, trauma and spinal cord injury, long-haul travel (>6 hours), varicose veins, antiphospholipid syndrome, myeloproliferative disorders, and polycythemia. Heritable risk factors include male sex, factor V Leiden mutation; prothrombin 20210A gene variant; antithrombin, protein C, and protein S deficiencies; and dysfibrinogenemias. In some patients, the cause of the thrombophilia may have both a heritable and an acquired component. These mixed causes include homocysteinemia; factor VII, VIII, IX, and XI elevation; hyperfibrinogenemia; and activated protein C resistance in the absence of factor V Leiden.11 There may be a synergistic effect when particular multiple inherited and acquired risk factors are present in the same patient.
Other patient-specific factors associated with venous thrombosis include the traditional cardiovascular risk factors of obesity, hypertension, and diabetes. VTE is more common in whites and African Americans than Asians and Native Americans.12,13 Certain gene variants (single nucleotide polymorphisms) are also associated with a mildly increased risk for VTE, and their presence may interact with other risk factors to increase the overall risk for venous thrombosis.14
Anatomic factors may also contribute to development of DVT. At the site where the right iliac artery crosses over the left iliac vein, the left iliac vein may become chronically narrowed predisposing to iliofemoral venous thrombosis, so-called May-Thurner syndrome. External compression of major veins by masses of various types can also lead to venous thrombosis.
Many cases of VTE are potentially preventable. Accordingly, in current clinical practice, preoperative VTE risk assessment is becoming increasingly common to identify patients at moderate and high risk. Scoring systems have been developed that take into account the number of VTE risk factors in an individual patient. These risk stratification scores, such as the Rogers score15 and Caprini score,16 provide individual patient risk stratification and recommendations for prophylactic anticoagulation. The ninth edition of the American College of Chest Physicians (ACCP) Guidelines for Prevention of VTE in Non-Orthopedic Surgical Patients acknowledges both the Rogers and Caprini scores and provides recommendations for VTE prophylaxis (Table 24-3). Orthopedic surgical patients are generally excluded from risk assessment scores because of the disproportionately increased risk of VTE in orthopedic surgery compared with the general and abdominopelvic surgery population.
LEVEL OF RISK | APPROXIMATE DVT RISK WITHOUT THROMBOPROPHYLAXIS (%) | SUGGESTED THROMBOPROPHYLAXIS OPTIONS |
---|---|---|
Very low risk General or abdominopelvic surgery | <0.5% (Rogers score <7; Caprini score 0) | No specific thromboprophylaxis Early ambulation |
Low risk General or abdominopelvic surgery | ~1.5% (Rogers score 7–10; Caprini score 1–2) | Mechanical prophylaxis |
Moderate risk General or abdominopelvic surgery | ~3.0% (Rogers score >10; Caprini score 3–4) | LMWH (at recommended doses), LDUH, or mechanical prophylaxis |
High bleeding risk | Mechanical prophylaxis | |
High risk General or abdominopelvic surgery | ~6% (Caprini score ≥5) | LMWH (at recommended doses), fondaparinux and mechanical prophylaxis |
High bleeding risk General or abdominopelvic surgery for cancer | Mechanical thromboprophylaxis Extended-duration LMWH (4 weeks) |
Early in the course of DVT development, venous thrombosis is thought to begin in an area of relative stasis, such as a soleal sinus vein or immediately downstream of the cusps of a venous valve in the axial calf veins. Isolated proximal DVT without tibial vein thrombosis is unusual. Early in the course of a DVT, there may be no or few clinical findings such as pain or swelling. Even extensive DVT may sometimes be present without signs or symptoms. History and physical examination are therefore unreliable in the diagnosis of DVT. In addition, symptoms and signs generally associated with DVT, such as extremity pain and/or swelling, are nonspecific. In large studies, DVT has been found by venography or DUS in ≤50% of patients in whom it was clinically suspected.17,18 Objective studies are therefore required to confirm a diagnosis of VTE or to exclude the presence of VTE.
Clinical symptoms may worsen as DVT propagates and involves the major proximal deep veins. Extensive DVT of the major axial deep venous channels of the lower extremity with relative sparing of collateral veins causes a condition called phlegmasia cerulea dolens (Fig. 24-4). This condition is characterized by pain and pitting edema with associated cyanosis. When the thrombosis extends to the collateral veins, massive fluid sequestration and more significant edema ensue, resulting in a condition known as phlegmasia alba dolens.19 The affected extremity in phlegmasia alba dolens is extremely painful and edematous and pale secondary to arterial insufficiency from dramatically elevated below lower knee compartment pressures. Both phlegmasia cerulean dolens and phlegmasia alba dolens can be complicated by venous gangrene and the need for amputation.
DUS is now the most commonly performed test for the detection of infrainguinal DVT, both above and below the knee, and has a sensitivity and specificity of >95% in symptomatic patients.3 DUS refers to the combination of real-time B-mode ultrasound with pulsed Doppler capability. For VTE detection, color flow imaging is an extremely useful adjunct in the evaluation of possible calf vein DVT and evaluation of intra-abdominal veins. DUS provides the ability to noninvasively visualize venous anatomy, detect occluded and partially occluded venous segments, and demonstrate physiologic flow characteristics using a mobile self-contained device.
In the supine patient, normal lower extremity venous flow is phasic (Fig. 24-5), decreasing with inspiration in response to increased intra-abdominal pressure with the descent of the diaphragm and then increasing with expiration as the diaphragm rises and intra-abdominal pressure decreases. When the patient is upright, the decrease in intra-abdominal pressure with expiration cannot overcome the hydrostatic column of pressure existing between the right atrium and the calf. Muscular contractions of the calf, along with the one-way venous valves, are then required to promote venous return to the heart. Flow also can be increased by leg elevation or compression and decreased by sudden elevation of intra-abdominal pressure (Valsalva maneuver). In a venous DUS examination performed with the patient supine, spontaneous flow, variation of flow with respiration, and response of flow to Valsalva maneuver are all assessed. From the common femoral through the popliteal vein, the primary method of detecting DVT with ultrasound is demonstration of the lack of compressibility of the vein with probe pressure on B-mode imaging. Normally, in transverse section, the vein walls should coapt with pressure. Lack of coaptation indicates thrombus. Calf vein thrombi are often best detected by abnormalities in color flow imaging.
The examination begins at the ankle and continues proximally to the groin. Each vein is visualized, and the flow signal is assessed with distal and proximal compression. Lower extremity DVT can be diagnosed by any of the following DUS findings: lack of spontaneous flow (Fig. 24-6), inability to compress the vein (Fig. 24-7), absence of color filling of the lumen by color flow DUS, loss of respiratory flow variation, and venous distention. Again, lack of venous compression on B-mode imaging is the primary diagnostic variable. Several studies comparing B-mode ultrasound to venography for the detection of femoropopliteal DVT in patients clinically suspected to have DVT report sensitivities of >91% and specificities of >97%.20,21 The ability of DUS to assess isolated calf vein DVT varies greatly, with sensitivities ranging from 50% to 93% and specificities approaching 100%.22,23
Impedance plethysmography (IPG) was the primary noninvasive method of diagnosing DVT before the widespread use of DUS but is infrequently used today. Changes in electrical resistance resulting from lower extremity blood volume changes are quantified. IPG is less accurate than DUS for the detection of proximal DVT, with 83% sensitivity in symptomatic patients. It is a poor detector of calf vein DVT.24
Iodine-125 fibrinogen uptake (FUT) is a seldom used technique that involves IV administration of radioactive fibrinogen and monitoring for increased uptake in fibrin clots. An increase of 20% or more in one area of a limb indicates an area of thrombus. FUT can detect DVT in the calf, but high background radiation from the pelvis and the urinary tract limits its ability to detect proximal DVT. It also cannot be used in an extremity that has recently undergone surgery or has active inflammation. In a prospective study, FUT had a sensitivity of 73% and specificity of 71% for identification of DVT in a group of symptomatic and asymptomatic patients.25 Currently, FUT is primarily a research tool of historic interest.
Venography is the gold standard to which other diagnostic modalities are compared. A small catheter is placed in a dorsal foot vein with injection of a radiopaque contrast agent. Radiographs are obtained in at least two projections. A positive study result is failure to fill the deep system with passage of the contrast medium into the superficial system or demonstration of discrete filling defects (Fig. 24-8). A normal study result virtually excludes the presence of DVT. In a study of 160 patients with a normal venogram followed for 3 months, only two patients (1.3%) subsequently developed DVT and no patients experienced symptoms of PE.26 Venography is not routinely used in clinical practice due to invasiveness and complication risk. It is still, however, frequently used in research studies evaluating DVT prophylaxis.
Once the diagnosis of VTE has been made, antithrombotic therapy should be initiated promptly. If clinical suspicion for VTE is high, it may be prudent to start treatment while the diagnosis is objectively confirmed. The goals of VTE treatment are the prevention of mortality and morbidity associated with PE and the prevention of the postthrombotic syndrome (PTS). Treatment regimens may include antithrombotic therapy, temporary or permanent vena cava filter placement, catheter-directed or systemic thrombolytic therapy, and operative thrombectomy.
Most often, antithrombotic therapy for VTE is initiated with IV or subcutaneous (SC) unfractionated heparin or SC low molecular weight heparin. Fondaparinux, a synthetic pentasaccharide, is sometimes also used as an alternative to heparin to initiate therapy. An oral vitamin K antagonist, usually sodium warfarin, is begun shortly after initiation of IV or SC therapy. Either SC or IV therapy is continued until effective oral anticoagulation with warfarin is achieved as indicated by an international normalized ratio (INR) ≥2 for 24 hours. A minimum of 5 days of heparin or fondaparinux therapy is recommended.27 Recently, the U.S. Food and Drug Administration (FDA) has also approved alternative oral anticoagulants for both treatment and prophylaxis for VTE.
Unfractionated heparin (UFH) binds to antithrombin via a specific 18-saccharide sequence. This increases antithrombin activity over 1000-fold. The antithrombin-heparin complex primarily inhibits factor IIa (thrombin) and factor Xa and, to a lesser degree, factors IXa, XIa, and XIIa of the coagulation cascade. In addition, UFH also binds to tissue factor pathway inhibitor, which inhibits the conversion of factor X to Xa, and factor IX to IXa. Finally, UFH catalyzes the inhibition of thrombin by heparin cofactor II via a mechanism independent of antithrombin.
UFH therapy is most commonly administered with an initial IV bolus of 80 units/kg. Weight-based UFH dosages have been shown to be more effective than standard fixed boluses in rapidly achieving therapeutic levels.28 The initial bolus is followed by a continuous IV drip at 18 units/kg per hour. The half-life of IV UFH ranges from 45 to 90 minutes and is dose dependent. The level of antithrombotic therapy should be monitored every 6 hours using the activated partial thromboplastin time (aPTT), with the goal range of 1.5 to 2.5 times control values. This should correspond with plasma heparin anti-Xa activity levels of 0.3 to 0.7 IU/mL.
Initial anticoagulation with UFH may also be administered SC, although this route is less commonly used. Adjusted-dose therapeutic SC UFH is initiated with 17,500 units, followed by 250 units/kg twice daily, and dosing is adjusted to an aPTT goal range similar to that for IV UFH. Fixed-dose unmonitored SC UFH is started with a bolus of 333 units/kg, followed by 250 units/kg twice daily.29
Hemorrhage is the primary complication of UFH therapy. The rate of major hemorrhage (fatal, intracranial, retroperitoneal, or requiring transfusion of >2 units of packed red blood cells) is approximately 5% in hospitalized patients undergoing UFH therapy (1% in medical patients and 8% in surgical patients).29 For patients with UFH-related bleeding complications, cessation of UFH is required, and anticoagulation may be reversed with protamine sulfate. Protamine sulfate binds to UFH and forms an inactive salt compound. Each milligram of protamine neutralizes 90 to 115 units of heparin, and the dosage should not exceed 50 mg IV over any 10-minute period. Side effects of protamine sulfate include hypotension, pulmonary edema, and anaphylaxis. Patients with prior exposure to protamine-containing insulin (NPH) and patients with allergy to fish may have an increased risk of hypersensitivity, although no direct relationship has been established. Protamine administration should be terminated if any side effects occur.
In addition to hemorrhage, heparin also has unique complications. Heparin-induced thrombocytopenia (HIT) results from heparin-associated antiplatelet antibodies (HAAbs) directed against platelet factor 4 complexed with heparin.30 HIT occurs in 1% to 5% of patients being treated with heparin.31,32 In patients with repeat heparin exposure (such as vascular surgery patients), the incidence of HAAbs may be as high as 21%.33 HIT occurs most frequently in the second week of therapy and may lead to disastrous venous or arterial thrombotic complications. Therefore, platelet counts should be monitored periodically in patients receiving continuous heparin therapy.
HIT is diagnosed based on previous exposure to heparin, platelet count less than 100,000, and/or platelet count decline of 50% following exposure. All heparin must be stopped and alternative anticoagulation initiated immediately to avoid thrombotic complications, which may approach 50% over the subsequent 30 days in affected individuals.34
Another complication of prolonged high-dose heparin therapy is osteopenia. Heparin-induced osteopenia results from impairment of bone formation and enhancement of bone resorption by heparin.
Low molecular weight heparins (LMWHs) are derived from the depolymerization of porcine UFH. Like UFH, LMWHs bind to antithrombin via a specific pentasaccharide sequence to expose an active site for the neutralization of factor Xa. However, LMWHs have fewer additional saccharide units. This results in less inactivation of thrombin (factor IIa). In comparison to UFH, LMWHs have increased bioavailability (>90% after SC injection), longer half-lives (approximately 4 to 6 hours), and more predictable elimination rates.
Most patients treated with weight-based once- or twice-daily SC LMWH injections do not require laboratory monitoring for anticoagulant effect, a distinct advantage over continuous IV infusions of UFH. Patients who do require monitoring include those with significant renal insufficiency, pediatric patients, obese patients greater than 120 kg, and pregnant patients. Monitoring may be performed using anti-Xa activity assays. The therapeutic anti-Xa goal range depends on the type of LMWH and the frequency of dosing. There are numerous LMWHs available, and the various preparations differ in their anti-Xa and anti-IIa activities. Treatment dosing for one LMWH therefore cannot be extrapolated for use with another. The anticoagulant effect of LMWHs may be partially reversed (approximately 60%) with protamine sulfate.
Numerous well-designed trials comparing SC LMWH with IV and SC UFH for the treatment of DVT have been critically evaluated in several meta-analyses and demonstrate a decrease in thrombotic complications, bleeding, and mortality with LMWHs.35,36,37 LMWHs also are associated with a decreased rate of HAAb formation and HIT (<2%) compared with UFH (at least in prophylactic doses).29 However, patients with established HIT also should not receive LMWHs because there is cross-reactivity between the drugs.38
A major benefit of LMWHs is that it allows outpatient treatment of VTE.39,40 In a randomized study comparing IV UFH and the LMWH nadroparin calcium,39 there was no significant difference in recurrent thromboembolism (8.6% for UFH vs. 6.9% for LMWH) or major bleeding complications (2.0% for UFH vs. 0.5% for LMWH). There was, however, a 67% reduction in mean days in the hospital for the LMWH group.
Fondaparinux currently is a synthetic pentasaccharide that has been approved by the FDA for the initial treatment of DVT and PE. Its five-polysaccharide sequence binds and activates antithrombin, causing specific inhibition of factor Xa. In two large noninferiority trials, fondaparinux was compared with the LMWH enoxaparin for the initial treatment of DVT and with IV UFH for the initial treatment of PE.41,42 The rates of recurrent VTE ranged from 3.8% to 5%, with rates of major bleeding of 2% to 2.6%, for all treatment arms. The drug is administered SC once daily with a weight-based dosing protocol: 5 mg, 7.5 mg,or 10 mg for patients weighing <50 kg, 50 to 100 kg, or >100 kg, respectively. The half-life of fondaparinux is approximately 17 hours in patients with normal renal function. There are rare case reports of fondaparinux-induced thrombocytopenia.43
Direct thrombin inhibitors (DTIs) include recombinant hirudin, argatroban, and bivalirudin. These antithrombotic agents bind to thrombin, inhibiting the conversion of fibrinogen to fibrin as well as thrombin-induced platelet activation. These actions are independent of antithrombin. The DTIs should be reserved for (a) patients in whom there is a high clinical suspicion or confirmation of HIT, and (b) patients who have a history of HIT or test positive for heparin-associated antibodies. In patients with established HIT, DTIs should be administered for at least 7 days, or until the platelet count normalizes. Warfarin may then be introduced slowly, overlapping therapy with a DTI for at least 5 days.44
Bivalirudin is approved primarily for patients with or without HIT who undergo percutaneous coronary intervention and is rarely used outside of that setting.
Commercially available hirudin is manufactured using recombinant DNA technology. It is indicated for the prophylaxis and treatment of patients with HIT. In patients with normal renal function, recombinant hirudin is administered as an IV bolus dose of 0.4 mg/kg, followed by a continuous IV infusion of 0.15 mg/kg per hour. The half-life ranges from 30 to 60 minutes. The aPTT is monitored, starting approximately 4 hours after initiation of therapy, and dosage is adjusted to maintain an aPTT of 1.5 to 2.5 times the laboratory normal value. The less commonly used ecarin clotting time is an alternative method of monitoring. Recombinant hirudin is eliminated via renal excretion, so dosage adjustments are required in patients with renal insufficiency.
Argatroban is indicated for the prophylaxis and treatment of thrombosis in HIT. It also is approved for patients with, or at risk for, HIT undergoing percutaneous coronary intervention. Antithrombotic prophylaxis and therapy are initiated with a continuous IV infusion of 2 μg/kg per minute, without the need for a bolus. The half-life ranges from 39 to 51 minutes, and the dosage is adjusted to maintain an aPTT of 1.5 to 3 times normal. Large initial boluses and higher rates of continuous infusion are reserved for patients with coronary artery thrombosis and myocardial infarction. In these patients, therapy is monitored using the activated clotting time. Argatroban is metabolized and excreted by the liver; therefore, dosage adjustments are needed in patients with hepatic impairment. There is no reversal agent for argatroban.
Vitamin K antagonists, which include warfarin and other coumarin derivatives, are the mainstay of long-term antithrombotic therapy in patients with VTE. Warfarin inhibits the γ-carboxylation of vitamin K–dependent procoagulants (factors II, VII, IX, and X) and anticoagulants (proteins C and S), resulting in formation of less functional proteins. Warfarin usually requires several days to achieve full effect because normal circulating coagulation proteins must first undergo their normal degradation. Factors X and II have the longest half-lives, in the range of 36 and 72 hours, respectively. A steady-state concentration of warfarin is usually not reached for 4 to 5 days.
Warfarin therapy is monitored by measuring the INR, calculated using the following equation:
where ISI is the international sensitivity index. The ISI describes the strength of the thromboplastin that is added to activate the extrinsic coagulation pathway. The therapeutic target INR range is usually 2.0 to 3.0, but the response to warfarin is variable and depends on liver function, diet, age, and concomitant medications. In patients receiving anticoagulation therapy without concomitant thrombolysis or venous thrombectomy, the vitamin K antagonist may be started on the same day as the initial parenteral anticoagulant, usually at doses ranging from 5 to 10 mg. Smaller initial doses may be needed in older and malnourished patients, in those with liver disease or congestive heart failure, and in those who have recently undergone major surgery.45
The recommended duration of warfarin antithrombotic therapy is stratified based on whether the DVT was provoked or unprovoked, whether it was the first or a recurrent episode, where the DVT is located, and whether malignancy or thrombophilia is present. Current ACCP recommendations for duration of warfarin therapy are summarized in Table 24-4.
CLINICAL SUBGROUP | ANTITHROMBOTIC TREATMENT DURATION |
---|---|
First episode DVT/transient risk/surgery | VKA or LMWH for 3 months |
First episode DVT/unprovoked | VKA or LMWH for 3 months Consider for long-term therapy if: • Proximal DVT • Minimal bleeding risk • Stable coagulation monitoring |
Distal DVT/unprovoked • Symptomatic • Asymptomatic and no risk factors for progression | VKA for 3 months Serial imaging in 2 weeks, if progression VKA for 3 months |
Second episode DVT/ unprovoked DVT and cancer | VKA for extended therapy LMWH for extended therapy over VKA |
In patients with proximal DVT, several randomized clinical trials have demonstrated that shorter-term antithrombotic therapy (4 to 6 weeks) is associated with a higher rate of VTE recurrence than 3 to 6 months of anticoagulation.46,47,48 In these trials, most of the patients with transient risk factors had a low rate of recurrent VTE, and most recurrences were in patients with continuing risk factors. The ACCP recommendation, therefore, is that 3 months of anticoagulation are sufficient to prevent recurrent VTE in patients with DVT occurring around the time of a transient risk factor (e.g., hospitalization, orthopedic or major general surgery).
In contrast to patients with thrombosis related to transient risk factors, patients with idiopathic VTE are much more likely to develop recurrence (rates as high as 40% at 10 years). In this latter group of patients, numerous clinical trials have compared 3 to 6 months of anticoagulation therapy with extended-duration warfarin therapy, both at low intensity (INR of 1.5 to 2.0) and at conventional intensity (INR of 2.0 to 3.0).49,50,51 In patients with idiopathic DVT, extended-duration antithrombotic therapy is associated with a relative reduction in the rate of recurrent VTE by 75% to >90%. In addition, conventional-intensity warfarin reduces the risk even further compared with low-intensity warfarin (0.7 events per 100 person-years vs. 1.9 events per 100 person-years) without an increase in bleeding complications.52
In patients with VTE in association with a hypercoagulable condition, the optimal duration of anticoagulation therapy is influenced more by the clinical circumstances at the time of the VTE (idiopathic vs. secondary) than by the actual presence or absence of the more common thrombophilic conditions. In patients with VTE related to malignancy, increasing evidence suggests that longer-term therapy with LMWH (up to 6 months) is associated with a lower VTE recurrence than treatment using conventional vitamin K antagonists.53,54
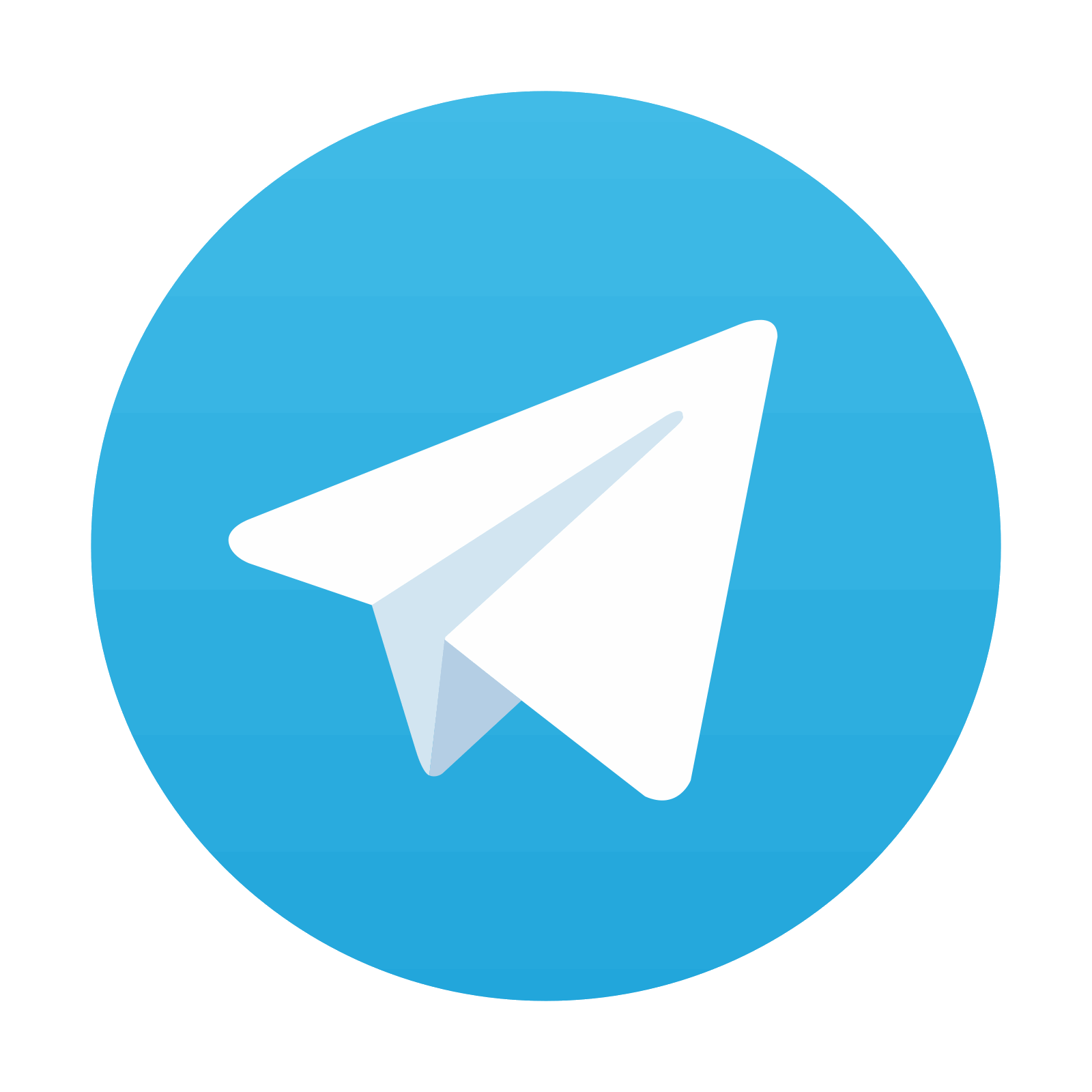
Stay updated, free articles. Join our Telegram channel
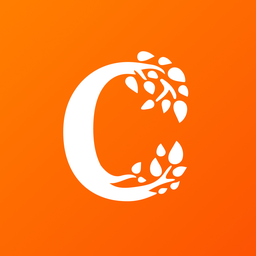
Full access? Get Clinical Tree
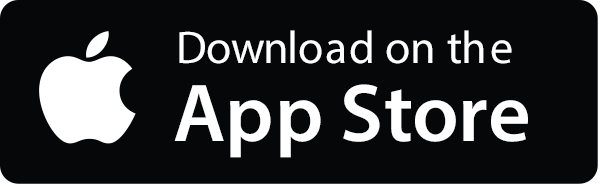
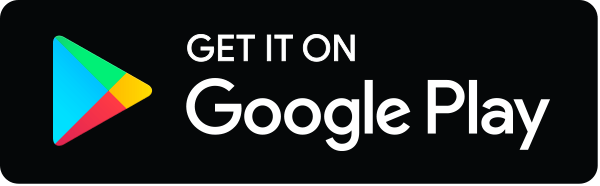
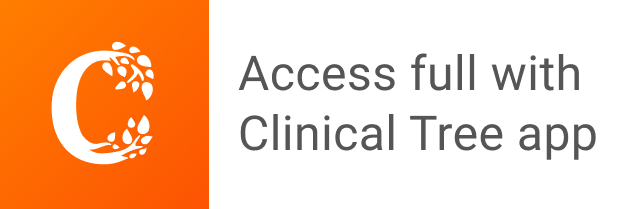