autonomic portions of the CNS. SA outflow during exercise is further modulated by changes in circulating levels of hormones and substrates and by the physical and chemical properties of the blood such as temperature, tonicity, pH, and oxygen and carbon dioxide tension. The SA system response is designed to address three major requirements of strenuous exertion: (1) the provision of oxygen and metabolic substrates to contracting muscle; (2) the need to dissipate excess heat generated by muscle contraction; and (3) maintenance of an adequate plasma volume. Training decreases SNS activity both during exercise and at rest (Figs. 3.2 and 3.3).
Substrate mobilization depends heavily on the fall in insulin secretion that accompanies fasting. This decrease in circulating insulin in conjunction with the small rise in E stimulates lipolysis and hepatic glucose output. Fibroblast growth factor 21 has also been proposed to stimulate lipolysis during fasting but this effect is controversial.
Suppression of the SNS during fasting subserves the useful function of diminishing energy expenditure, a conservative mechanism that would prolong survival during fasting.
The physiologic mechanisms linking dietary intake with SNS activity have been well worked out: decreased insulin-mediated glucose uptake and metabolism in neurons of the ventromedial hypothalamus stimulates an inhibitory pathway to tonically active SNS neurons in the brainstem (Fig. 3.6). During fasting, the small fall in glucose and the larger fall in insulin decrease insulin-mediated glucose metabolism in the insulin-sensitive neurons of the ventromedial hypothalamus. This decrease in glucose metabolism stimulates an inhibitory pathway from the hypothalamus to the tonically active SNS neurons in the brainstem resulting in suppression of central sympathetic outflow. This is an example of regulation by descending inhibition, a fundamental principal elaborated by the famous British neurophysiologist Sir Charles Sherrington. Evidence for this sequence is summarized in Table 3.1.
![]() FIGURE 3.4. Fasting decreases NE turnover in heart. (Modified From Young JB, Landsberg L. Suppression of sympathetic nervous system during fasting. Science. 1977;196:1473-1475.) |
TABLE 3.1 Insulin-Mediated Glucose Uptake in the VMH Regulates SNS Activity in Response to Diet | ||
---|---|---|
|
effect even when added as excess calories (Fig. 3.9). Overfeeding a mixed (“cafeteria”) diet also activates the SNS (Fig. 3.10). The link between diet and the SNS involves insulin and glucose and is the converse of that described above for fasting (Fig. 3.6). During feeding or overfeeding, the small rise in glucose and the large rise in insulin increases insulin-mediated glucose metabolism in the hypothalamic neurons thereby decreasing activity in the inhibitory pathway to the brainstem with release of the tonically active SNS neurons and an increase in central sympathetic outflow. These dietary effects occur in humans as well as in small mammals. The stimulatory effect of euglycemic insulin infusions on SNS activity in humans is shown in Figure 3.11.
![]() FIGURE 3.7. Effect of sucrose on cardiac NE turnover. (From Young JB, Landsberg L. Stimulation of the sympathetic nervous system during sucrose feeding. Nature. 1977;269:615-617.) |
does not stimulate the SNS (Fig. 3.9) and that low protein diets are extremely stimulatory (Fig. 3.12).
TABLE 3.2 Counter-Regulatory Effects of Epinephrine | |
---|---|
|
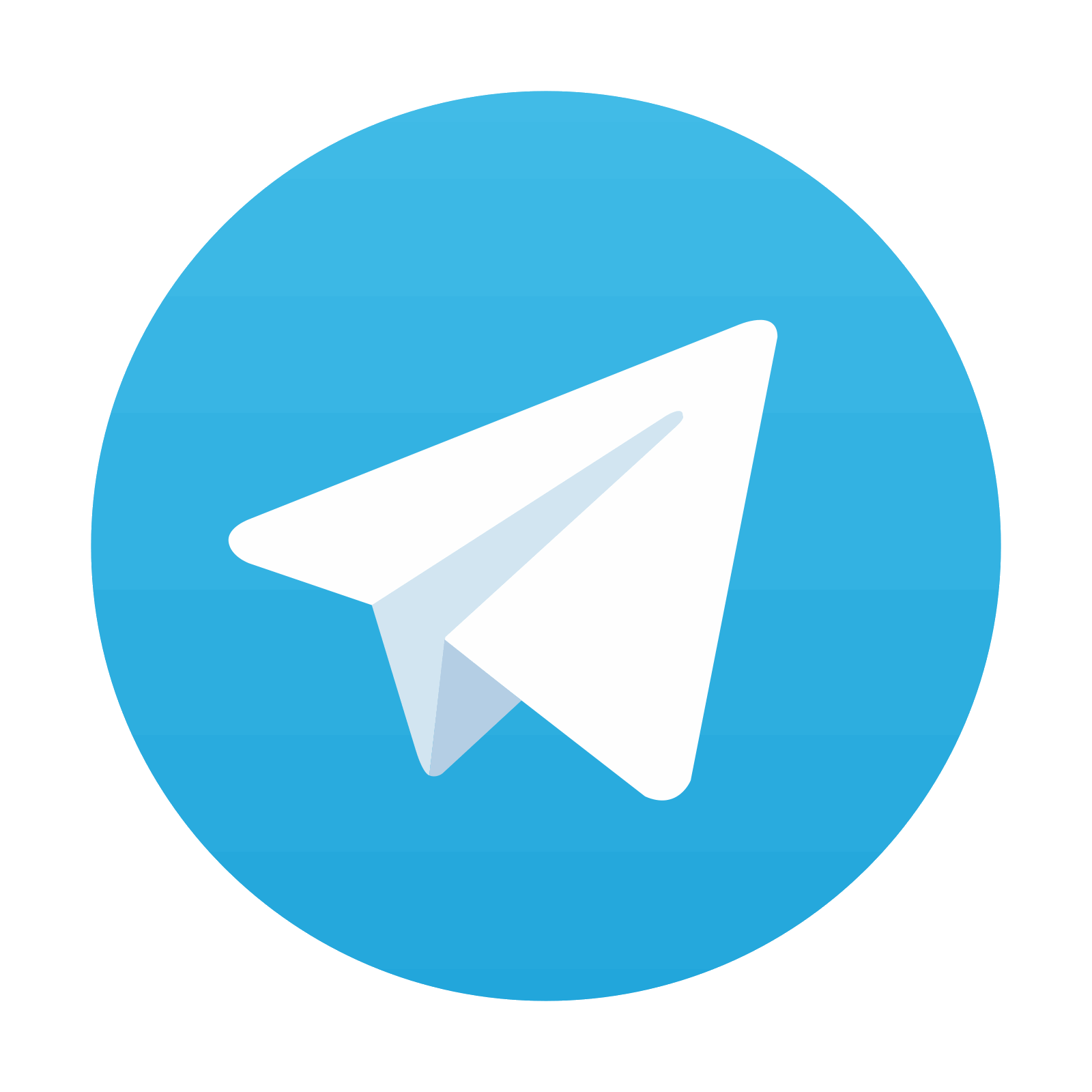
Stay updated, free articles. Join our Telegram channel
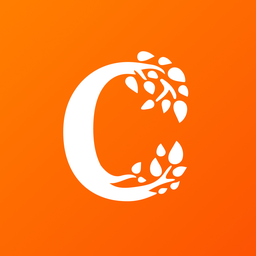
Full access? Get Clinical Tree
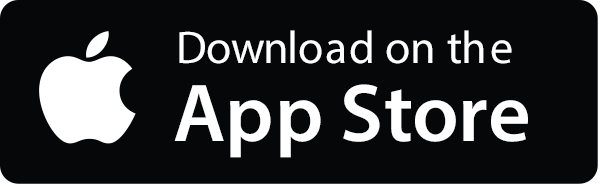
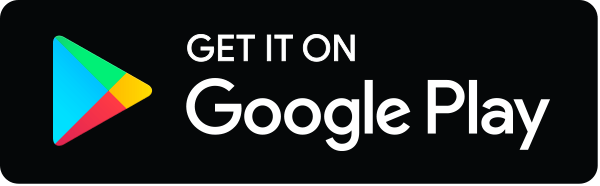