Normal heart and aorta, gross
In this anterior view, the heart and great vessels are shown: aortic root (◼), aortic arch (□), thoracic aorta (+), and pulmonic trunk (∗). The anterior interventricular (descending) coronary artery (▲) extends down the anterior surface of the heart. Note the smooth epicardial surface with yellowish epicardial adipose tissue.

Normal heart, gross ▶
On the posterior surface of the heart is the right coronary artery (∗), which becomes the posterior descending artery (▲). Note the right ventricle (◼), left ventricle (□), right atrium (+), and left atrium (♦). A small amount of fluid between the epicardial surface shown and the enclosing pericardium (not shown) provides gliding motion with cardiac contraction.

Normal heart, gross
The left lateral aspect of the heart displays the left atrial appendage (+) and left ventricle (□). The circumflex artery (∗) gives off marginal branches (▲). The normal adult heart weighs approximately 250 to 300 g in women and 300 to 350 g in men. The left ventricle, averaging 1.3 to 1.5 cm in thickness, produces the cardiac output that serves the systemic circulation. The right ventricle, 0.3 to 0.5 cm in thickness, works under a lower pressure to supply the pulmonary circulation. The ejection fraction (EF) is the amount of blood ejected from the left ventricle (LV) with each heartbeat and is normally more than 55% of filled volume. An EF can be calculated with an echocardiogram, a left ventriculogram done during cardiac catheterization, or a nuclear medicine study called a MUGA. The method of calculating the EF involves tracing the dimensions of the LV at the end of its contraction period (systole) and at the end of its relaxation period (diastole).

Normal myocardium, microscopic
Normal cardiac muscle in longitudinal section is composed of a syncytium of myocardial fibers (cardiac myocytes) with centrally located nuclei. Cardiac myocytes are a form of striated muscle with units called sarcomeres that contain the contractile proteins myosin and actin. Faint dark pink intercalated discs (▲) traverse myocytes, forming mechanical and electrical couplings through gap junctions. Red blood cells are seen in single file in the numerous capillaries between the myocardial fibers. Ultrastructurally there are numerous mitochondria and abundant myoglobin to power a lifetime of heart beats.

Normal conduction system, microscopic
The cardiac conduction system, difficult to observe histologically in humans, consists of specialized myocytes that conduct electrical impulses more readily than surrounding myocardial fibers. The neural differentiation of myocytes in this cross-section of atrioventricular node is highlighted in brown-orange color by this S100 immunohistochemical stain. The initial pacemaker of the heart is the sinoatrial node in the right atrium, and the specialized conducting myocytes spread excitation pulses, leading to a wave of depolarization through the atria, which is then conducted through the atrioventricular node and down the bundle of His into the ventricles.

Normal coronary artery, microscopic
The major coronary branches have a large central lumen to supply blood to the heart. The intima (▲) is normally thin and indistinct. The media (◼) with smooth muscle forms the bulk of the artery. The adventitia (+) outside the media merges with surrounding epicardial adipose and connective tissue. Distally, major coronary arterial branches bifurcate to smaller branches. Shown is a distal coronary artery branch with a prominent lumen that is adjacent to myocardium at upper right. Such arteries anchored by myocardium are less likely to have turbulent blood flow and less likely to develop atherosclerosis. Atherosclerosis tends to develop in the proximal portions of major coronary arteries.

Normal aortic valve, gross
The aortic valve, similar to the other semilunar valve—the pulmonic valve—has three thin, delicate cusps. The coronary artery orifices (↓) can be seen just above the aortic valve cusps. The endocardium of the left ventricle is smooth, beneath which can be seen the red-brown myocardium. The aorta above this valve displays a smooth intimal surface with no atherosclerosis, typical for persons with a healthy lifestyle.

Normal tricuspid valve, gross
The leaflets of the atrioventricular valves (mitral and tricuspid) are thin and delicate. Similar to the mitral valve, the leaflets shown here have thin chordae tendineae (↑) that tether the leaflet margins to the papillary muscles of the ventricular wall below the valve. The right atrium is seen above the valve. Endocardial surfaces are smooth and translucent.

Normal heart, CT image
This normal chest CT scan in “bone window” shows the right lung (∗), left lung (□), right atrium (□), right ventricle (♦), left atrium (+), left ventricle (▲), aortic root (†), and descending aorta (×) in the upper chest. The lungs, filled with air, have greatly decreased attenuation (less brightness) consistent with “air density” for x-rays. Bright contrast material fills the heart chambers. The chest wall is normal, with bright bone in the ribs and vertebral column.

Brown atrophy, gross
Virtually all cardiac diseases eventually lead to an enlarged heart. Here is a rare example of “brown atrophy” in which the heart is small, with chocolate-brown myocardium. In this condition, there is excessive lipochrome (lipofuscin) deposition within myocardial fibers. Aging and malnutrition may favor this process, a pronounced form of cellular autophagocytosis. Antioxidants may protect against such injury. In the normal aging process, the amount of lipofuscin increases within myocardial fiber cytoplasm, but not to the degree seen here.

Lipofuscin, microscopic
The stippled, finely granular, intracytoplasmic, golden-brown pigment (↑) that lies primarily in a perinuclear location within these myocytes is lipofuscin (lipochrome) pigment. This “wear-and-tear” pigment represents the remnants of long-term autophagocytosis and cell remodeling accompanying free radical formation and lipid peroxidation. The small amounts seen here, which increase with aging, produce no significant pathologic effect. Aging is not a disease and is not a cause for organ failure. Healthy diet and exercise are key to maintaining cellular function.

Cardiac hypertrophy, gross
Note prominent concentrically thickened left ventricle from hypertrophy (◼). The number of myocardial fibers does not increase, but their size can increase in response to an increased workload, leading to the marked thickening of the left ventricular wall and septum. Increased pressure load from systemic hypertension is the most common cause. An increased volume load from aortic regurgitation can also lead to hypertrophy. Some degree of cardiac chamber dilation also accompanies ventricular failure. There is an ongoing relative decreased capillary density, increased fibrous tissue, and synthesis of abnormal proteins that predispose to heart failure.

Cardiomegaly, x-ray
This posteroanterior (PA) chest radiograph shows marked cardiomegaly, with the left heart border (+) extending far into the left chest. Ordinarily, the cardiac shadow occupies approximately half the distance across the chest from one rib margin to the other. The most common causes for an enlarged heart include ischemic heart disease and systemic hypertension. Intrinsic disease of the myocardium may produce a cardiomyopathy with enlargement. Pulmonary hypertension can lead to cor pulmonale with initial right-sided enlargement. Eventually, failure of the left or right ventricle leads to failure of the opposite ventricle, and there is more likely to be global cardiac enlargement with any long-standing disease affecting the heart.

Cardiomegaly, CT image
Note the large size of this diseased heart, with the left heart filling much of the left chest cavity, in this patient with cardiomegaly from systemic hypertension. Right (♦) and left (◼) ventricles are also dilated as compensation with increasing heart failure. In this “lung window,” the interstitial markings within the lungs appear more prominent from vascular congestion.

Heart failure and effusions, MRI
This T2-weighted MR image of a neonate in coronal view shows a bright pericardial effusion (+) around the heart. There are also ascites with bright fluid (♦) around the intra-abdominal organs in the peritoneal cavity. Such effusions can occur with hydrops (generalized edema), and heart failure from causes such as anemia, infection, and congenital cardiac anomalies.

Heart failure and effusions, ultrasound
This ultrasound image of a neonate of shows a large area of diminished echogenicity (♦) within the pericardial cavity around the heart. This effusion is a fluid collection that is most often a serous transudate with congestive heart failure, also leading to perinatal hydrops.

Patent foramen ovale, gross
The right panel shows a metal probe lifting the interatrial septum secundum to reveal an abnormal opening—a probe patent foramen ovale in an adult heart. Normally, left atrial pressure keeps this foramen closed, but if right atrial pressure increases with pulmonary hypertension (acutely with pulmonary embolus), the probe patent foramen may open and even allow a thromboembolus (→), seen in the left panel , to go from right to left. This is a rare paradoxical embolus , so called because a thromboembolus arising within the venous circulation can travel to the systemic circulation.

Atrial septal defect (ASD), gross
This large (♦) ASD led to a left-to-right shunt and pulmonary hypertension with increased pulmonary arterial pressures that eventually caused reversal and right-to-left shunt, resulting in marked right ventricular hypertrophy, a complication known as Eisenmenger complex . The examiner’s finger (lower left) holds a markedly thickened right ventricular free wall below the tricuspid valve, and the finger (upper right) holds the interventricular septum below the mitral valve. Approximately 90% of ASDs such as this are secundum defects. Primum defects account for 5% of ASDs and are often associated with an anterior mitral leaflet cleft. The remainder are sinus venosus defects near the entrance of the superior vena cava.

Ventricular septal defect (VSD), gross
This large VSD (♦) lies just below the aortic valve. A third of VSDs are isolated defects without accompanying anomalies, but many diagnosed perinatally or in infancy are part of multiple anomalies. This VSD involves the membranous septum, as are 90% of VSDs, whereas 10% occur in the muscular interventricular septum. Approximately half of small VSDs may eventually close. A large VSD with a significant left-to-right shunt leads to cardiac failure, and if not corrected surgically, the shunt leads to pulmonary hypertension with cor pulmonale and eventual reversal to a right-to-left shunt (Eisenmenger complex). VSDs increase the risk for infectious endocarditis.

Atrial septal defect (ASD) and ventricular septal defect (VSD), gross ▶
The heart is opened on the left side with the left ventricular free wall reflected superiorly to reveal two defects (←) including both an ASD above and a muscular VSD below. Such small defects may not produce significant left-to-right shunting, but they do increase the risk for infective endocarditis, and a holosystolic murmur may often be audible on auscultation of the chest. A muscular VSD is more likely to close spontaneously than a membranous VSD.

Endocardial cushion defect, gross
A severe defect is shown in which there is only a single large atrioventricular valve, viewed from the atrial aspect superiorly, that separates a single ventricle from a single atrium. This patient was able to survive with this two-chambered heart because a small amount of residual interventricular septum provided some directional separation to flow of oxygenated and unoxygenated blood, and because of pulmonic stenosis, which protected the lungs from the shunting. (This is an explanted heart from a cardiac transplantation procedure, so most of the atria are not present.)

Aortic coarctation, gross
This adult aorta opened longitudinally reveals a region of narrowing (↑). There was increased turbulence that led to increased atherosclerosis. Overall, males are affected twice as often as females, but coarctation is a common feature of monosomy X (Turner syndrome). Coarctation is categorized in relation to the ductus arteriosus. The preductal form with proximal aortic tubular hypoplasia is also known as the infantile form because of symptoms appearing early in life. The postductal form usually becomes symptomatic later in life, with findings related to diminished blood flow to lower extremities, but hypertension in the upper body.

Coronary atherosclerosis, gross
A minimal amount of coronary atherosclerosis, with a few scattered yellow lipid plaques (↓), is seen on the intimal surface of the opened coronary artery traversing the epicardial surface of a heart, with adipose tissue. The degree of atherosclerosis here is not great enough to cause significant luminal narrowing, but could be the harbinger of worse atherosclerosis to come, if plaques continue to enlarge. Atherosclerosis is initiated with endothelial damage and inflammation with leukocyte elaboration of cytokines, such as TNF, IL-6, and IFN-γ, and serum markers like CRP. This process is promoted by uptake of increased circulating oxidized LDL cholesterol into macrophages.

Coronary atherosclerosis, gross
These cross-sections of the left anterior interventricular (descending) coronary artery show atherosclerosis with more pronounced luminal narrowing at the leftmost, more proximal portion of this artery. Atherosclerosis is generally worse near the coronary arterial origin, in the first few centimeters, where turbulent blood flow is greater. This turbulent flow over many years promotes endothelial injury that favors inflammation with insudation of lipids to promote formation of atheromas. With lifestyle modifications, this process is reversible.

Coronary atherosclerosis, microscopic
The coronary artery in the left panel is narrowed by 60% to 70%, on the verge of producing angina, which could then be precipitated by transient vasoconstriction. Acute coronary syndromes from marked ischemia are more likely to occur when luminal narrowing reaches 70%. The coronary artery in the right panel has more severe occlusion, with evidence for previous thrombosis and organization of the thrombus leading to recanalization, such that there are only three small residual arterial lumens remaining for blood flow.

Coronary atherosclerosis, microscopic
This atheromatous plaque shows endothelial denudation with plaque disruption and overlying thrombus (∗) formation from platelet aggregation. Note the composition of the plaque base with pale foam cells, thin cholesterol clefts, and red areas of hemorrhage. Such a plaque complicated by rapid overlying thrombus formation can lead to an acute coronary syndrome resulting in an ischemic cardiac event. The first sign of ischemic heart disease may be angina pectoris, a symptom complex characterized by recurrent acute episodes of substernal or precordial chest pain. Unstable angina will not be accompanied by an increase in serum troponin. Occlusive coronary atherosclerosis increases the risk for subsequent myocardial infarction.

Coronary thrombosis, gross
Thrombus formation is a serious complication of coronary atherosclerosis, shown here within thickened arterial walls with yellow-tan plaques that narrow the arterial lumen. The dark red thrombotic (♦) occludes this anterior descending coronary artery, opened longitudinally. The thrombotic occlusion leads to ischemia or infarction of the myocardium supplied by the artery, typically ST-elevation myocardial infarction with transmural infarction. One possible outcome of coronary thrombosis is sudden death. Other complications include ongoing arrhythmias, angina, and congestive heart failure.

Coronary thrombosis, microscopic
This recent thrombus, formed of red blood cells along with paler platelets and fibrin, nearly occludes the remaining small lumen of this coronary artery already narrowed from severe atherosclerosis. Note the fibrointimal proliferation (∗) with cholesterol clefts. Endothelial damage with platelet activation promotes thrombosis. A small dose of aspirin taken each day helps reduce platelet function, making the platelets less “sticky” and less prone to participate in thrombotic events.

Myocardial infarction (MI), gross
The interventricular septum is sectioned to reveal an extensive acute MI. The dead muscle is tan-yellow, with a surrounding hyperemic border (←). This appearance is characteristic of an infarction that is 3 to 7 days old. The serum marker troponin I is released from damaged myofibers and begins to increase 3 to 4 hours after the initial ischemic event. The serum troponin level is proportionate to the amount of infarction, and it remains elevated in the serum for 10 to 14 days. Serum myoglobin can be increased starting 3 hours after MI, but it is not specific for myocardium.

Myocardial infarction (MI), gross
This axial section reveals a large transmural MI involving the anterior left ventricular wall and interventricular septum in the distribution of the left anterior descending coronary artery. Note the yellowish area (♦) of necrosis surrounded by a hyperemic border. Radionuclide imaging would show decreased uptake into this region. Echocardiography would show diminished ventricular wall motion and decreased left ventricular ejection fraction. EKG changes include ST-segment elevation (STEMI) followed by T wave inversion and development of Q waves. Serum troponin would be elevated.

Myocardial infarction (MI), gross
In cross-section, the point of apical left ventricular free wall rupture of myocardium is shown at the arrow . In this case, MI 3 weeks prior accounts the ventricular wall thinning seen here, and a subsequent MI occurred, ruptured through an already thinned ventricular wall 3 days later. The mitral valve with chordae tendineae and the papillary muscles appear normal here. Rupture is most likely to occur 3 to 7 days after a transmural infarction, when the necrotic muscle is soft, and before any significant amount of organization with ingrowth of capillaries and fibroblasts has occurred.

Myocardial infarction (MI), microscopic
The earliest histologic change seen with acute MI during the first 24 hours is contraction band necrosis. These myocardial fibers are beginning to lose cross-striations, and the central nuclei are not clearly visible in most of these myocytes. Note the many irregular, darker pink, wavy contraction bands (∗) extending across the fibers. Serologic markers for infarction include nonspecific myoglobin and the more specific markers of cardiac muscle injury: troponin I. Use of thrombolytic agents, percutaneous transluminal coronary angioplasty, and coronary arterial bypass grafting are methods to help restore blood flow and prevent further damage.

Myocardial infarction (MI), microscopic
This trichrome stain shows the appearance of an early acute MI, less than 1 day old, with prominent reddish contraction band necrosis (round red blood cells lie within capillaries). Coagulative necrosis with karyolysis has led to loss of the nuclei. If the area of infarction remains small, the MI may be “silent” without signs or symptoms and detectable only by electrocardiography or by serum cardiac muscle enzyme elevation. The myocardial irritability after an MI leads to electrical conduction disturbances with arrhythmias such as sinus bradycardia, heart block, asystole, and ventricular fibrillation. ST-segment elevation (STEMI) on EKG is more likely with complete coronary occlusion, and non-STEMI (NSTEMI) with partial occlusion and reduced myocardial blood flow relative to oxygen demand.

Myocardial infarction (MI), microscopic
This early acute MI is 1 to 2 days old. There is loss of cross-striations, and some contraction bands (∗) are seen. The cardiac fiber nuclei have undergone karyolysis and are no longer visible. Some neutrophils (+) are beginning to infiltrate this necrotic myocardium. The loss of nuclei represents an irreversible form of cellular injury. Reperfusion of such damaged muscle may lead to increased production of toxic free radicals that can potentiate further myocardial damage. Percutaneous coronary intervention or thrombolytic therapy to treat acute coronary thrombosis is beneficial soon after the initial occlusion.

Myocardial infarction (MI), microscopic
This early acute MI is 2 to 3 days old. There is increasing infiltration of neutrophils (▲) into the infarcted myocardium. With ongoing coagulative necrosis, the outlines of myofibers remain, but their nuclei have undergone dissolution. At this point the serum troponin marker remains elevated. The extent of infarction determines residual ventricular function. A large infarct may severely reduce left ventricular ejection fraction and lead to acute congestive heart failure with pulmonary congestion and edema. Electrical dysfunction with arrhythmia may occur. Focal pericarditis may complicate transmural infarction.
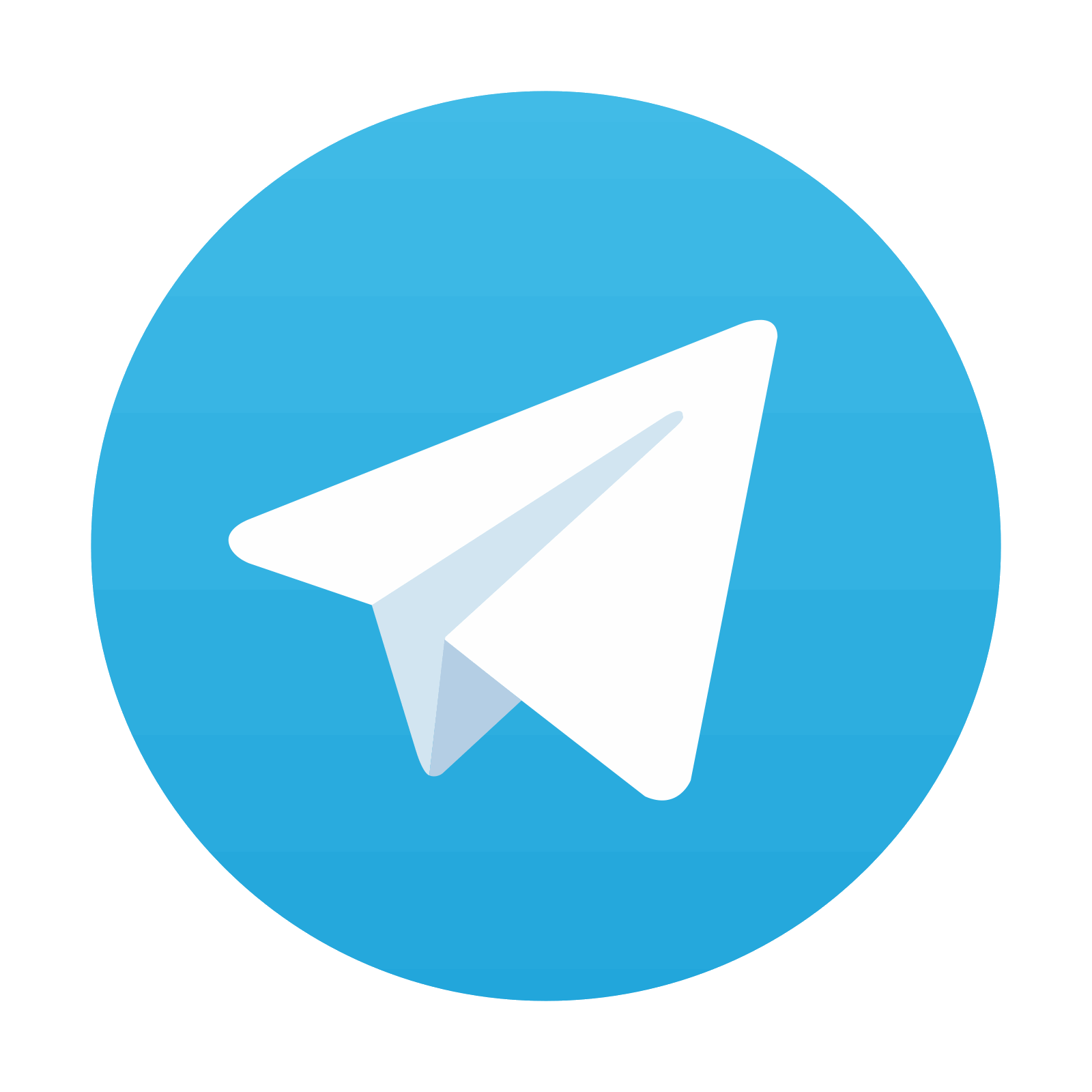
Stay updated, free articles. Join our Telegram channel
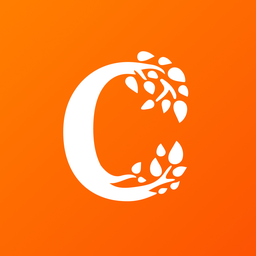
Full access? Get Clinical Tree
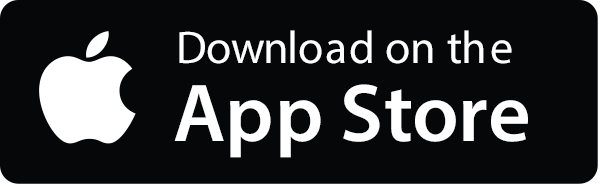
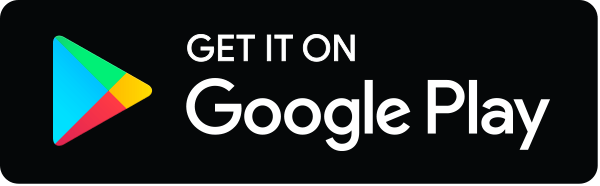
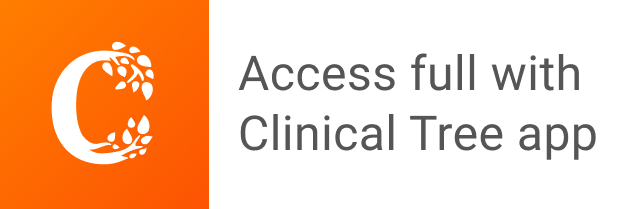