The Gastric Acid Pump
The gastric H,K ATPase exchanges H+ for K+ at equal stoichiometry. Even though the outward and inward parts of the transport cycle are electrogenic, pump transport is electroneutral and does not generate a transmembrane potential. The general transport process that the α subunit of this pump catalyzes is shown in the following figure. More detail has been gleaned from homology analysis with the structure of the Ca2+ ATPase and by site-directed mutagenesis to elucidate the binding region of the K+-competitive inhibitor—the 1,2α imidazo-pyridine SCH28080—and from binding of the proton pump inhibitors (PPIs) to different cysteines accessible from the luminal surface of the catalytic subunit.
Reaction pathway of the H,K ATPase
The Na,K ATPase exchanges intracellular sodium ions for extracellular potassium ions, the gastric ATPase H3O+ for extracellular potassium, and the sarcoplasmic or endoplasmic reticular Ca2+ pump two cytoplasmic Ca2+ for 2H+ in the sarcoplasmic reticulum. In the outward reaction transporting Na+, H+, or Ca2+, the catalytic subunit is phosphorylated by ATP, and in the inward reaction, the phosphoenzyme is dephosphorylated by K+ or H+ (in the case of the sarcoplasmic reticulum Ca2+ ATPase). To achieve uphill transport at a significant rate, there has to be a decrease in binding-site affinity on the side of the membrane from which the ion is transported and reorientation of this site toward the side to which the ion is transported.
A conceptual model for this H+ outward and K+ inward transport that is supported experimentally is that the transported ion enters the membrane domain from one side and binds to a site with relatively high affinity with phosphorylation to provide the transporting conformation. Then the membrane domain closes to occlude the ion to provide the occluded conformation. Another conformational change then opens the membrane domain to allow the ion to be removed from the cytoplasm to escape from the other side of the membrane from
a lower-affinity state of the ion-binding site. Binding of the countertransported ion induces the converse changes in affinity and conformation, the ion binding with relatively high affinity to the outside face, occluding, and releasing from the low-affinity state to the cytoplasm.
a lower-affinity state of the ion-binding site. Binding of the countertransported ion induces the converse changes in affinity and conformation, the ion binding with relatively high affinity to the outside face, occluding, and releasing from the low-affinity state to the cytoplasm.
The experiment on the Na+ pump that established occlusion took advantage of the fact that this enzyme forms a phosphorylated intermediate. In the presence of 100 mM of Na+ and ATP, phosphoenzyme is formed at a constant rate. Various other cations can substitute for K+ in the dephosphorylation reaction, such as Rb+, Cs+, or NH4+. These are K+ surrogates. If the concentration of these cations is set to give equal rates of dephosphorylation, but the enzyme turns over at a different rate, the rate-limiting step must be subsequent to dephosphorylation and before rephosphorylation. It was postulated that this step was the unbinding of the occluded cation present in the membrane domain of the dephosphorylated enzyme.
This hypothesis was then shown to be correct by demonstrating the binding of 86Rb+ to the pump in the presence of ouabain and showing that the addition of ATP resulted in deocclusion. Similar binding of cation has also been demonstrated for the sarcoplasmic reticulum Ca2+ ATPase and the gastric H,K ATPase, establishing the mechanistic similarity of these enzymes.
The scheme of their reaction is shown in the figure illustrating the kinetic steps for the H,K ATPase. This shows that the enzyme exists in three major conformations, with ion-binding sites facing inward [the “in” conformation (E1)], ion-binding sites facing outward [the “out” conformation (E2)], and ion sites occluded (the “occ” conformation). The transition between these conformations is driven by ATP binding, transphosphorylation, and dephosphorylation.
Kinetics of the H,K ATPase
Kinetic studies on the H,K ATPase have defined the reaction steps shown in the figure illustrating the H+ and K+ transport steps. Understanding of the overall catalytic cycle of this ion pump has facilitated a mechanistic description of the process of acid secretion by the stomach. From this derives our understanding of stimulation of acid secretion and the processes inhibited by the PPIs.
The key structural change in the ion-binding site, apart from sidedness, is the size of the ion it can accommodate. In the “in” conformation, the smaller hydronium ion, H3O+, is accommodated. In the “out” conformation, the smaller ion is weakly bound compared to the larger, partially hydrated K+. Experimental evidence for the reaction cycle described above has been obtained using rapid kinetic analysis of the rate of formation and destruction of phosphoenzyme generated from 32P-ATP at different pH and potassium concentrations.
The rate of formation of the phosphoenzyme and the K+-dependent rate of breakdown are sufficiently fast to allow the phosphoenzyme to be an intermediate in the overall ATPase reaction. The initial step is the reversible binding of ATP to the enzyme in the absence of added K+ ion, followed by a Mg2+– (and proton-) dependent transfer of the terminal phosphate of ATP to the catalytic subunit (E1-P•H+). The Mg2+ remains occluded until dephosphorylation. Increasing the hydrogen ion concentration on the ATP-binding face of the vesicles accelerates phosphorylation, whereas increasing the potassium ion concentration inhibits phosphorylation. Increasing the hydrogen ion concentration reduced K+ inhibition
of the phosphorylation rate. Decreasing the hydrogen ion concentration accelerated dephosphorylation in the absence of K+, and K+ on the luminal surface accelerated dephosphorylation. Increasing K+ concentrations at constant ATP decreased the rate of phosphorylation, and increasing ATP concentrations at constant K+ concentration accelerated ATPase activity and increased the steady-state phosphoenzyme level. Therefore, inhibition by the alkali cations is due to cation stabilization of a dephosphorylated E1 form at a cytosolically accessible cation-binding site. Occlusion was demonstrated directly by showing 86Rb binding to the enzyme at a low temperature that was released by the addition of ATP.
of the phosphorylation rate. Decreasing the hydrogen ion concentration accelerated dephosphorylation in the absence of K+, and K+ on the luminal surface accelerated dephosphorylation. Increasing K+ concentrations at constant ATP decreased the rate of phosphorylation, and increasing ATP concentrations at constant K+ concentration accelerated ATPase activity and increased the steady-state phosphoenzyme level. Therefore, inhibition by the alkali cations is due to cation stabilization of a dephosphorylated E1 form at a cytosolically accessible cation-binding site. Occlusion was demonstrated directly by showing 86Rb binding to the enzyme at a low temperature that was released by the addition of ATP.
The addition of K+ to the enzyme-bound acylphosphate results in a two-step dephosphorylation. The faster initial step is dependent on the concentration of K+. The second phase of EP breakdown is accelerated in the presence of K+, but at K+ concentrations exceeding 500 μM, the rate becomes independent of K+ concentration. This shows that two forms of EP exist. The first form, E1P, is K+ insensitive and converts spontaneously to E2P, the K+-sensitive form. ATP binding to the H,K ATPase occurs in both the E1 and the E2 states, but with a lower affinity in the E2 state. As for the Na+ pump, various cations, such as Rb+, Cs+, NH4+, and Tl+, can act as K+ substitutes or surrogates. As will be seen later, the design of K+-competitive inhibitors of the H,K ATPase takes advantage of the surrogate properties of NH4+. At higher concentrations of K+, the rate of hydrolysis slows as the removal of K+ from the E1 conformation of the pump becomes rate limiting.
Membrane potential and the H,K ATPase
The gastric H,K ATPase is electroneutral, in contrast to the Na,K ATPase, because the number of H+ ions exported is the same as the number of K+ ions imported. However, each half of the reaction cycle generates an equal but opposite membrane potential as the ion traverses the membrane domain.
The H+ for K+ stoichiometry of the H,K ATPase was reported to be one or two per ATP hydrolyzed. The H+:ATP ratio was independent of external KCl and ATP concentrations. If care is taken to measure initial rates in tight vesicles, the ratio is 1ATP:2H:2K, at pH 6.1. Because at full pH gradient the stoichiometry must fall to 1ATP:1H:1K, this pump displays a variable stoichiometry. This can be explained if at least two carboxylic amino acids are involved in ion binding and one of them stays protonated as the pH of the acid space falls to below the negative logarithm of the acid ionization constant (pKa) of one of the carboxylic acid residues. Since the ion binding sites face into the center of the ion transport pathway that is hydrophilic, the pKa is likely to be between 3.0 and 4.0. Hence, the stoichiometry will fall to 1:1 at a pH of approximately 3.0.
2D structure of the gastric H,K ATPase
Eventually, a complete description of the mechanism of the H,K ATPase will include a detailed three-dimensional structure of this enzyme with the changes seen on binding ATP, phosphorylation, and ion binding and transport, as have been obtained for the SERCA ATPase.
The catalytic subunit is similar in general structure to that of other mammalian small cation P-type ATPases. Composed of 1,034 or 1,035 amino acids, it has a large cytoplasmic domain, a connecting stalk or energy transduction domain, a transmembrane domain, and a small extracytoplasmic domain. The β subunit has an N-terminal cytoplasmic sequence of approximately 60 amino acids, a transmembrane domain of approximately 30 amino acids, and a C-terminal domain containing approximately 200 amino acids with seven N-linked glycosylation sites. Although the function of the pumps that have been sequenced is usually known, it is not possible by inspection of the sequence to predict which ion is transported by which sequence. In the 1980s, when complementary deoxyribonucleic acid (cDNA) and, hence, amino acid sequences became available, there was hope that linear sequence would provide mechanistic clues as to protein function. Amino acid sequencing has enabled enormous advances in the fields of phenotyping cells, analyzing families of proteins, and defining interacting proteins and signature sequences but has not fully enabled functional or mechanistic analysis. Biochemical, molecular, and structural features are necessary in addition to be able to call the function of a protein sequence.
The catalytic α and β subunit amino acid sequence
The primary sequences of the α subunits deduced from cDNA have been reported for several species such as pig, rat, rabbit, and human. The hog gastric H,K ATPase, a subunit sequence deduced from its cDNA, consists of 1,034 amino acids and has a molecular weight (MWt) of 114,285 Da. The sequence, based on the known N-terminal amino acid sequence, is one less than the cDNA-derived sequence and begins with glycine. The degree of conservation among the α subunits is extremely high (over 97% identity). The human gastric H,K ATPase gene has 22 exons and encodes a protein of 1,035 residues, including the initiator methionine residue (MWt = 114,047). These H,K ATPase α subunits show high homology (˜60% identity) with the Na,K ATPase catalytic α subunit. The distal colon K+ ATPase α subunit, H,K α2, has also been sequenced and shares 75% homology with both the H,K and Na,K ATPases.
The amino acid sequence derived from the cDNA for the hog enzyme is shown in the figure. The sequences for this enzyme and of the sodium pump are 75% homologous. Regions of identity are the phosphorylation consensus sequence and the ATP-binding domain; many of the cytoplasmic regions have high homology compared to the transmembrane regions. It is remarkable that, even with the detailed knowledge of the amino acid sequence, the function of the protein must be measured experimentally and cannot be predicted.
The β subunit
The primary sequences of the β subunits have been reported for rabbit, hog, rat, mouse, and human enzymes and contain approximately 290 amino acids. The hydropathy profile of the β subunit is less ambiguous than that of the α subunit. There is one membrane-spanning region predicted by the hydropathy analysis, which is located at the region between positions 38 and 63 near the N terminus. Tryptic digestion of the intact gastric H,K ATPase produces only one small cleavage of the N-terminal segment of the β subunit on sodium dodecyl sulfate (SDS) gels. Wheat germ agglutinin (WGA) binding of the β subunit is retained due to the N-linked sugar residues. These data indicate that most of the β subunit is extracytoplasmic and glycosylated. When lyophilized hog vesicles are cleaved by trypsin followed by reduction, a small, nonglycosylated peptide fragment is seen on SDS gels with the N-terminal sequence AQPHYS, which represents the C-terminal region beginning at position 236 in the pig sequence. This small fragment is found neither after trypsinolysis of intact vesicles nor in the absence of reducing agents. A disulfide bridge must therefore connect this cleaved fragment to the β subunit containing the carbohydrates. The C-terminal end of the disulfide is at position 262. This leaves little room for an additional membrane-spanning α helix. Hence, the β subunit has only one membrane-spanning segment.
Region of association of the α and β subunits
The β subunit of both the Na,K and H,K ATPases is necessary for targeting the complex from the endoplasmic reticulum to the plasma membrane. It also stabilizes a functional form of both the gastric H,K and Na,K ATPases. The region of association of the two subunits helps explain this functional association, and this association is apparently vital for stable membrane folding of the α subunit. The β subunit, however, can traffic to the plasma membrane in the absence of the α subunit.
In the case of the Na,K ATPase, the last 161 amino acids of the α subunit are essential for effective association with the β subunit. Further, the last four or five C-terminal hydrophobic amino acids of the Na+-pump β subunit are essential for interaction with the α subunit, whereas the last few hydrophilic amino acids are not. Expression of the Na+-pump α subunit, along with the β subunit of either the sodium or proton pump in Xenopus oocytes, has shown that the β subunit of the gastric proton pump can act as a surrogate for the β subunit of the sodium pump for membrane targeting and 86Rb+ uptake. This implies homology in the associative domains of the β subunits of the two pumps. The H,K ATPase α subunit requires its α subunit for efficient cell-surface expression. Expression of chimers of the α subunits of the Na,K and Ca2+ ATPases showed that the C-terminal half of the α subunit assembled with the β subunit.
To specify the region of the α subunit associated with the β subunit, the tryptic digest was solubilized using nonionic detergents such as NP-40 or C12E8. These detergents allow the holoenzyme to retain ATPase activity. The tryptic fragments were then adsorbed to a WGA-affinity column. After elution of the peptides not associated with the β subunit binding to the WGA column, elution of the β subunit with 0.1 N acetic acid eluted almost quantitatively the M7/loop/M8 sector of the α subunit. These data show that this region of the α subunit is tightly associated with the β subunit such that nonionic detergents are unable to dissociate it from the β subunit.
If tryptic digestion is carried out in the presence of K+
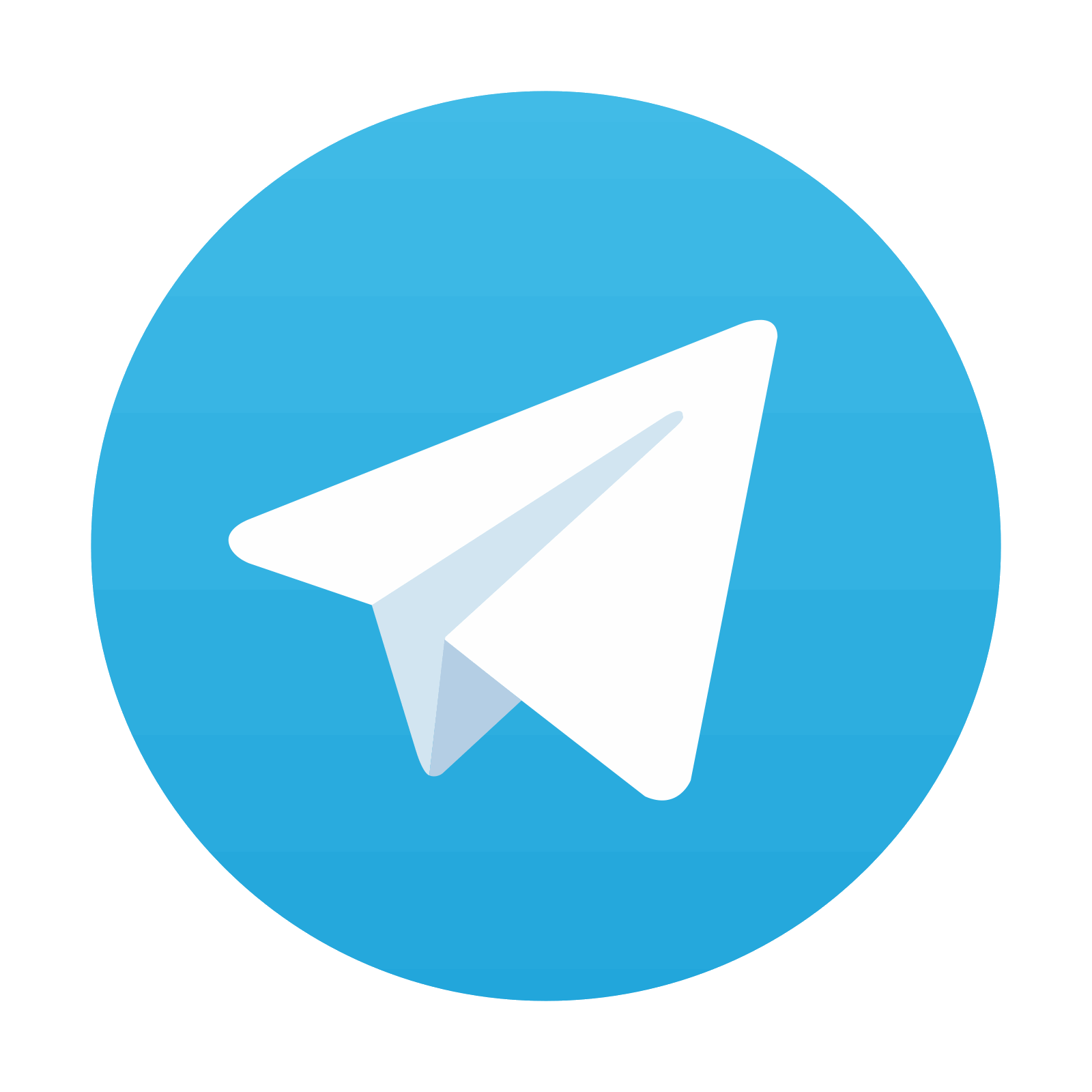
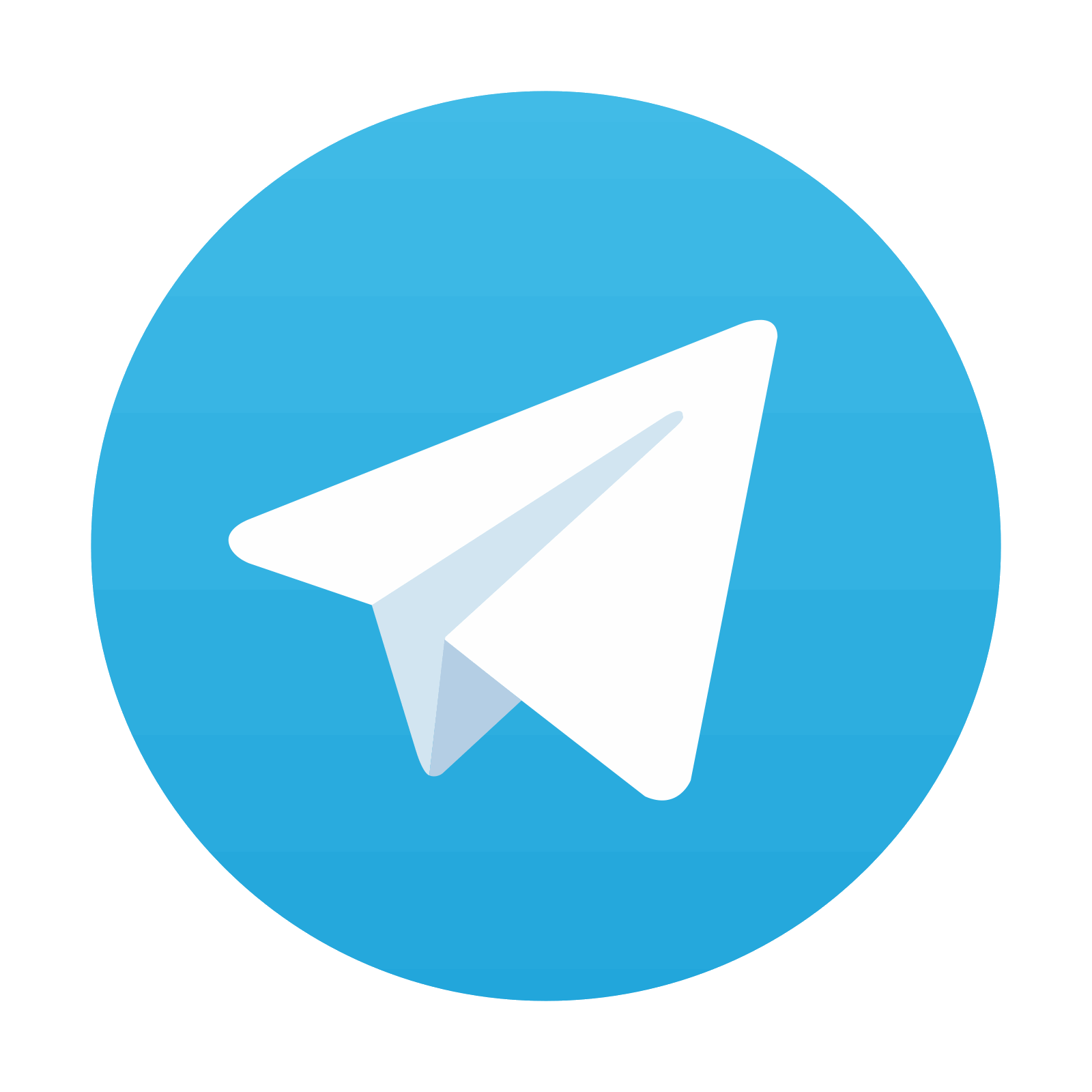
Stay updated, free articles. Join our Telegram channel
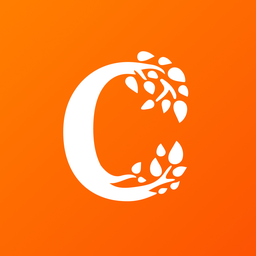
Full access? Get Clinical Tree
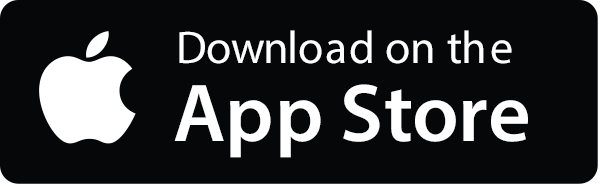
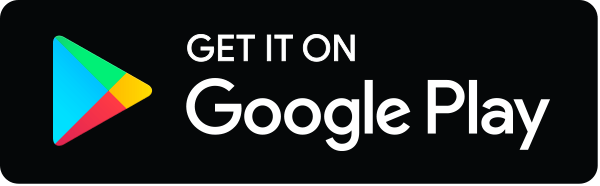
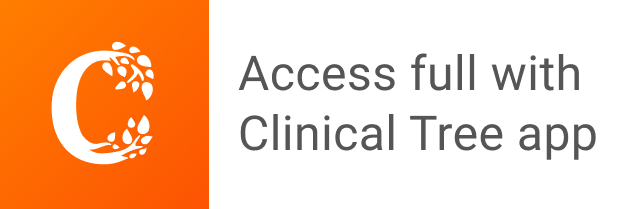