Normal brain, gross
The superior aspect at the vertex of an adult brain is shown here with the central sulcus (♦) between the right and left hemispheres. Note the pattern of gyri and sulci beneath the thin, filmy meninges (pia and arachnoid layers; the overlying dura has been removed). The rolandic fissure with the precentral gyrus (■) (motor cortex) and the postcentral gyrus (□) (somesthetic cortex) are seen here. The normal adult brain weighs 1100 to 1700 g.

Normal brain, gross
The lateral view of the brain reveals the frontal lobe (◄), parietal lobe (▼), temporal lobe (▲), occipital lobe (►), cerebellum (×), and brainstem (+). Note the sylvian fissure (♦) separating the frontal lobe from the temporal lobe.

Normal brain, gross
At the base of the brain can be seen the inferior frontal lobes (►), temporal lobes (▲), pons (+), medulla oblongata (♦), cerebellar hemispheres (□), and occipital lobes (◄).

Normal brain, gross
A sagittal section through the midline of the brain reveals the frontal lobe (►), parietal lobe (▼), and occipital lobe (◄). The genu (■) and the splenium (□) of the corpus callosum appear above the third ventricle, divided by the thin membrane, the septum pellucidum (+). The midbrain ( † ), pons (♦), and medulla oblongata (×) form the brainstem. The aqueduct of Sylvius connects the third ventricle to the fourth ventricle (▲). The fourth ventricle lies below the cerebellum (∗) and above the medulla.

Normal brain, gross
This coronal section through the center of the brain reveals the mammillary bodies (▲), globus pallidus (+), putamen (♦), caudate nucleus (◄), lateral ventricles (□), corpus callosum (▼), and hippocampus (×). This section is not completely symmetric (as is the case with many CT scans and MR images), so the amygdala (►) appears on just one side.

Normal brain, gross
This axial (transverse) section through the brain reveals the frontal lobe (►), caudate nucleus (+), anterior commissure (×), putamen (■), globus pallidus (□), medial (▲) and lateral (▼) geniculate nuclei, temporal lobe ( † ), parietal lobe (∗), and anterior vermis (◄) of the cerebellum.

Normal neocortex, microscopic
The neocortex (gray matter) of the cerebral hemispheres has six layers that are microscopically indistinct with H&E staining. Beneath the pia-arachnoid on the far left is an outer plexiform (♦) layer with nerve cells arranged horizontally. Next is the outer granular layer ( † ) containing small pyramidal neurons. Next is the outer pyramidal cell layer (■) with medium-sized pyramidal neurons. Below this is the inner granular layer (+) of larger pyramidal neurons. Beneath this is the inner pyramidal layer (□) of larger pyramidal neurons. The innermost cortical layer is the polymorphous layer (×), which lacks pyramidal cells. Beneath the cortex is the white matter (∗).

Normal hippocampus, microscopic
The normal appearance of the hippocampus is seen here at low magnification. Hippocampus consists of “paleocortex” with three layers: polymorphic (■), pyramidal neuronal (♦), and molecular (∗) layers. The granule cell layer (►) of the dentate gyrus is present.

Normal cerebellum, microscopic
The normal appearance of the cerebellum is seen here at low magnification with immunostain for calcineurin, highlighting the large brown Purkinje cells with their extensive arborizing dendritic network into the pale staining molecular layer above, like that drawn by Prof. Ramón y Cajal. The blue-colored granule layer is below.

Normal brain, microscopic
This immunohistochemical stain for intermediate filaments known as glial fibrillary acidic protein highlights astrocytes with their prominent processes that extend between neurons. At least one process either extends to the overlying pia or surrounds a capillary to form the blood-brain barrier along with endothelial cells and pericytes, a diffusion barrier that prevents the influx of most compounds from blood to brain. Pericytes encircle endothelial cells, providing structural support and aiding in controlling blood flow. Tight junctions between endothelial cells form the selective diffusion barrier. Astrocytic foot processes closely adherent to small vessels aid in the induction and maintenance of the tight junction barrier.

Cerebral edema, gross
This coronal section of cerebrum shows marked compression with effacement of lateral ventricles (▲) and flattening of gyri (◄) from extensive bilateral cerebral edema. This patient was climbing a 5000-m mountain peak and ignored the warning sign of a persistent, worsening headache. The hypoxia led to endothelial damage with an increase in intracellular edema (cytotoxic edema). In contrast, vasogenic edema results from disruption of the blood-brain barrier by inflammation or neoplasia, or generalized ischemia, so the two forms often overlap. In either case, edema increases intracranial pressure, with risk for herniation.

Cerebral edema, gross
The cortical surface beneath the meninges of this brain with cerebral edema shows widened, flattened gyri (∗) with narrowed sulci (♦). Disruption of the blood-brain barrier by inflammation or neoplasms can lead to vasogenic edema with fluid leakage into intercellular spaces. Ischemia results in cytotoxic edema from direct cell injury and an increase in intracellular fluid. Both conditions can be localized or diffuse. When there is extensive edema, generally both injury patterns are present. There are no intracranial lymphatics to scavenge the excess fluid, but glial cells may assist in fluid movement.

Cerebral edema, MRI
An abscess (♦) with inflammation that compromises the blood-brain barrier to produce surrounding edema appears bright in this T2-weighted axial MR image of the brain at the level of the orbits. Fluid appears bright, as in the globe of the eye (▼) and in the lateral ventricles (▲) compressed by the mass effect of the edema, with shift of the midline to the right (toward the bottom of the image). The brain tissue with edema appears brighter as well. This edema is most pronounced in the white matter.

Herniation, gross
The inferior aspect of this brain shows medial temporal lobe (uncinate) herniation on the left. Note the resulting compression of the left side of the midbrain. The medial temporal lobe tissue is pushed beneath the tentorium (▼), called transtentorial herniation. This can occur either by a mass effect or from edema on the ipsilateral side of the brain. Impingement upon cranial nerves can occur, particularly prone to cause oculomotor (CN III) nerve palsy.

Herniation with Duret hemorrhages, gross
Medial temporal lobe (uncal) herniation (▼) has produced a deep groove on the right medial temporal lobe (uncus) caused by pressure against the tentorium (not present in this image). This has caused bleeding (▲) into the upper pons, known as Duret hemorrhage . When the herniated tissue pushes the brainstem further down into the posterior fossa and stretches and tears the small perforating vessels into the pons and midbrain, this type of secondary brainstem bleeding occurs. When the degree of herniation is pronounced, hemiparesis ipsilateral to the side of the herniation may be caused by compression of the contralateral cerebral peduncle.

Cerebellar tonsillar herniation, gross
Acute brain swelling above the tentorium or within the posterior fossa can force posterior fossa contents downward toward the foramen magnum, and this can often produce herniation of the cerebellar tonsils into the foramen magnum. Note the cone shape of the cerebellar tonsils (◄) around the medulla seen here. Compression of the medulla compromises brainstem centers controlling respiration and cardiac activity, leading to death from herniation.

Hydrocephalus, gross
Note the marked dilation (■) of these cerebral ventricles. The adjacent white matter may develop interstitial edema. Hydrocephalus can be due to a lack of absorption of cerebrospinal fluid (CSF), called communicating hydrocephalus, or to an obstruction to flow of CSF, called noncommunicating hydrocephalus . Hydrocephalus can be a long-term complication of infection, such as a basilar meningitis, which leads to scarring that obstructs CSF outflow through the foramina of Luschka and Magendie. Inflammation with scarring of arachnoid granulations at the vertex may diminish CSF absorption.

Hydrocephalus, CT image
This axial view shows enlarged lateral ventricles. This condition has been treated by placing two shunts (the linear bright objects). Temporary ventricular shunts can be placed acutely to relieve hydrocephalus, or a permanent shunt can be placed with routing of the fluid to the peritoneum, where it is resorbed and recycled. The choroid plexuses normally produce 0.5 of CSF per day, but at any time, approximately 100 to 150 mL of CSF fills the ventricular system. This CSF is an ultrafiltrate of plasma that provides a “shock absorber” and cleansing function for the brain. The CSF circulates through the ventricles and spinal canal. CSF is normally reabsorbed at the arachnoid granulations at the vertex of the brain.

Hydrocephalus ex vacuo, gross
This coronal section shows a moderate degree of cerebral atrophy, which is more severe in the temporal lobe (◄) regions. The sylvian fissures appear enlarged. Note the moderate dilation of the cerebral ventricles, including the temporal horns, secondary to parenchymal loss. The condition called hydrocephalus ex vacuo results from brain parenchymal loss (with atrophy) to cause compensatory ventricular enlargement. There is no intrinsic abnormality of cerebrospinal fluid production, flow, or absorption. This patient had Alzheimer disease leading to the cerebral cortical atrophy.

Anencephaly, gross
The most dramatic form of neural tube defect (NTD) is anencephaly. This malformation of the caudal portion of the neural tube is marked by failure of formation of the fetal cranial vault. The unprotected brain cannot form when exposed to amniotic fluid. The eyes (▼) appear proptotic (right panel) because of the lack of a forehead for perspective. The reddish tissue (◄) at the base of the brain (left panel) represents the area cerebrovasculosa, a residual disorganized mass of glial, meningeal, and vascular tissue. A small amount of brainstem tissue may be spared, providing for short survival after birth. The open defect predisposes to postnatal infection. Supplementing the maternal diet with folate before and during early pregnancy can help to reduce the risk for NTDs, which otherwise occur at a rate of approximately 1 to 5 per 1000 live births.

Meningomyelocele, gross
Neural tube defect (NTDs) result from improper embryonic neural tube closure. The most minimal defect is called spina bifida, in which the posterior arch of a vertebral body fails to fuse completely, but the defect is not open to the skin. Open NTDs extend to and through the thin layer of skin covering the defect and can include meningocele, with just meninges protruding through the defect. The meningomyelocele (▲) seen here is large enough to allow meninges and a portion of spinal cord to protrude through the defect, with loss of spinal cord function from that level down. Open defects predispose to CNS infection. Such defects can be suspected prenatally by finding an elevated maternal serum or amniotic fluid α-fetoprotein and acetylcholinesterase.

Encephalocele, gross
This severe neural tube defect (NTD) is an occipital encephalocele with brain tissue extending through a posterior skull defect and forming a sac-like structure (▼) covered by skin. The cranial vault is present but appears flattened because there is less brain tissue developing within the cranial cavity. The herniated brain tissue is not functional. A frontoethmoidal encephalocele appears as a mass along the nose; a basal skull encephalocele appears as an oropharyngeal mass. NTDs may be an isolated anomaly or part of malformation syndromes.

Rachischisis, gross
The most common form of neural tube defect (NTD) is failure of closure of the posterior (caudal) neural tube, leading to spinal dysraphism, or spina bifida. This condition may not be severe, with only a portion of the vertebral arches missing (spina bifida occulta), but with an overlying skin covering, evidenced by a small skin dimple that may contain a tuft of hair over the site of the defect. A more severe form of NTD, known as rachischisis, is shown here with a large open defect (▲) involving the upper thoracic and cervical vertebrae, along with absence of the occipital bone. Because the cranial vault (►) also appears to be absent, the condition seen here is best termed craniorachischisis .

Exencephaly, gross
This is the rarest form of neural tube defect, known as exencephaly . Note here the absence of the cranial vault, but the presence of brain tissue (▼) covered only by thin meninges. In this condition, all or part of the fetal cranial vault may be missing, but there is still enough tissue covering the brain to allow its development in utero. Exencephaly is most likely to occur in conjunction with an early amnion disruption sequence, or limb–body wall complex, often with amnionic bands involving the head.

Iniencephaly, gross
This variation of neural tube defect (NTD) results from lack of proper formation of occipital bones, along with a short neck and defect of the upper spinal cord. The head is tilted back (▲), giving the appellation “stargazer” to infants with this rare condition. An encephalocele or a rachischisis typically accompanies iniencephaly. In any form of NTD, the amount of CNS tissue that fails to form or is disrupted or secondarily affected by infection or trauma determines the extent of neurologic deficits, including motor and sensory loss. Bowel and bladder function can be lost with low defects; NTDs in the thoracic or lumbar regions may lead to paraplegia; high NTDs involving the spinal cord can lead to quadriplegia.

Polymicrogyria, gross
The developmental abnormality in this newborn brain seen from the left lateral aspect, with loss of normal contours of the cerebral hemispheric convolutions, is called polymicrogyria. Note the numerous small gyral bumps (▲) over the lateral surface of this hemisphere. These are not separated by sulci microscopically. In this anomaly, the gyri are abnormally fused together, with entrapment of meningeal tissue, and there are no more than four cortical layers instead of the normal six-layered neocortex. This entire brain is too small (microcephaly). Genetic, teratogenic, and material factors may play a role in such an appearance.

Lissencephaly, gross
This coronal section of a child’s brain shows markedly diminished gyral development (◄), along with marked thickening of the underlying cerebral cortex. The hippocampi (►) and the deep gray matter structures appear normal. Lissencephaly (agyria) here led to severe developmental delay. 17p13.3 deletion with loss of the LIS1 gene occurs in the rare Miller-Dieker syndrome with agyria, seizures, and mental retardation. In normal fetal brain development, gyri first become visible about 20 weeks’ gestation, so lissencephaly before 20 weeks is expected. After 20 weeks, gyral development continues to term in an orderly manner.

Agenesis of the corpus callosum, gross
In this coronal section of brain, no corpus callosum can be seen. Only the small bundles of callosal fibers, the Probst bundles (▲) remain laterally, and the cingulate gyri above them appear displaced downward. This is one of the most common anomalies seen in the CNS; it may occur as an isolated event or in association with other anomalies. It may be complete or partial. It may be completely asymptomatic and detectable only with specialized testing. The much smaller but adaptable anterior commissure can assume the duties of the missing corpus callosum, part of the plasticity of neural development.

Alobar holoprosencephaly, gross
The “alobar” form of holoprosencephaly seen here has only a single large ventricle (♦) and no attempt at formation of separate cerebral hemispheres. Holoprosencephaly is often accompanied by the failure of fetal facial midline structures to form properly, with midline facial defects, such as cleft lip, cleft palate, and cyclopia, typically associated with a chromosomal abnormality, such as trisomy 13. It can also be seen with maternal diabetes mellitus or occur sporadically. Some cases are associated with mutation of the human Sonic Hedgehog gene.

Semilobar holoprosencephaly, gross
The fetal skull is opened here at autopsy to reveal the semilobar form of holoprosencephaly, so named because there is a small cleft (▲) representing partial development of the cerebral hemispheres. There is no apparent gyral pattern here because this stillborn fetus was less than 20 weeks’ gestation, and the lissencephaly is appropriate for this gestational age. Holoprosencephaly is a grave condition with little or no brain function.

Arnold-Chiari I malformation, gross
This sagittal section shows the cerebellar tonsils (►) herniated downward over the cervical spinal cord. There is enlargement of lateral ventricles from hydrocephalus (♦), thought to be secondary to poor flow of cerebrospinal fluid out the foramina of Luschka and Magendie, owing to the compression of brain tissue within the posterior fossa. Headache and neck pain are common. Cerebellar signs like ataxia may be present. Motor and sensory problems may occur with accompanying syringomyelia. Neurosurgical repair can be performed.

Arnold-Chiari I malformation, MRI
This MR image of the brain in midline sagittal view shows a small posterior fossa, and the cerebellar tonsils (◄) herniate through the foramen magnum. The third ventricle (♦) is enlarged, and the lateral ventricles may be enlarged. This is a milder form of Chiari malformation, and these patients may not have any symptoms. Patients affected by neurologic complications can be treated surgically to decompress the cervicomedullary junction and restore normal cerebrospinal fluid flow.

Arnold-Chiari II malformation, gross
In the less common Chiari II malformation, there is also a small posterior fossa with both tonsillar (►) and brainstem herniation. This more severe malformation shows kinking (+) of the medulla over the cervical cord, and there is a syrinx (▲) within the cervical spinal cord as well. The collicular plate (▼) extends upward (called tenting ), and there is invariably an associated hydrocephalus. Brainstem dysfunction often manifests in infancy and childhood. A lumbar meningomyelocele is usually present.

Dandy-Walker malformation, gross
Features include agenesis or hypoplasia of the cerebellar vermis, cystic dilatation of the fourth ventricle, and enlargement of the posterior fossa. There is replacement of the vermis by a midline cyst lined by ependyma and contiguous with leptomeninges, forming a roofless enlarged fourth ventricle (▲) seen here in the midline and below the cerebellar hemispheres laterally. There may be dysplasias of brainstem nuclei as well. Hydrocephalus can be present due to atresia of foramina.

Hydromyelia, gross, compared with syringomyelia, microscopic
The cross-sections of cervical spinal cord (left panel) show prominent dilation (♦) of the central canal known as hydromyelia . This dilated canal is lined by ependyma. With syringomyelia (right panel) there is a slit-like cavity (■) extending across the spinal cord, which often cuts across the anterolateral system tracts, leading to loss of upper extremity pain and temperature sensation. Extension of the lesion superiorly into the medulla is termed syringobulbia . This lesion is most often associated with Chiari malformations. Other associations include spinal cord trauma, inflammation, or intraspinal tumors.

Porencephaly, gross
Shown here is a large defect (∗) involving a cerebral hemisphere of a child, with an abnormal opening through the cerebral hemisphere between the ventricular system and the subarachnoid space. This condition can be developmental or secondary to an insult, thought to be vascular, early in utero that destroys the tissue and produces the defect. A familial form is related to COL4A1 gene mutations with fragile blood vessels. In contrast, schizencephaly is a developmental disorder of neuronal migration producing a cerebrospinal fluid–filled cleft lined by gray matter that extends across the entire cerebral hemisphere, from the ventricular surface to the pial surface of the brain.

Hydranencephaly, CT image
All that remains of this fetal brain in the supratentorial compartment are the basal ganglia (■), the inferior occipital lobes (∗), and a small remnant of frontal lobe (▼) adjacent to the falx cerebri (▲). This is a more dramatic example of a developmental or secondary insult in utero in which a large portion of the developed brain is destroyed. Most of the cerebral hemispheres are missing. The consequence is a fluid-filled space covered by meninges, mimicking hydrocephalus, but the size of the head may not be increased, because cerebrospinal fluid pressure is not increased.

Hydranencephaly, gross
This is an example of unilateral hydranencephaly, observed at autopsy of a child, with the cranial cavity opened superiorly. The cranial cavity is almost empty on the left. There is a small remnant of the left occipital lobe (■), and there is a bump of tissue representing the residual left basal ganglia (♦). The right cerebral hemisphere, shown here reflected laterally, is formed properly. An ischemic event in utero may have led to this finding.

Cerebral palsy, MRI
This developmental disorder of motor function is present from infancy or early childhood, probably resulting from a vascular accident with localized infarction occurring prenatally or intrapartum. The actual event may go unrecognized until a motor problem, such as spasticity, dystonia, or paresis is noted early in child development. In this case, the child had impaired motion, particularly of extensor muscles, on the left. Seen here is asymmetric mild loss of white matter, basal ganglia, and thalamus on the right with unilateral ventricular ex vacuo dilation (♦). Cerebral palsy is nonprogressive, and the childhood brain has an amazing plasticity with ability to “rewire” itself, minimizing the deficit over time.

Germinal matrix hemorrhage, gross
This coronal section of brain from a 25-week gestational age premature infant shows a germinal matrix hemorrhage (▲) that occurred shortly after birth. The germinal matrix is a highly vascularized area bordering the caudate nucleus and thalamus that is very sensitive to injury from variations in blood pressure (lack of autoregulation) and hypoxia. The risk for hemorrhage is greatest for premature infants born at 22 to 32 weeks’ gestation, with a peak at 28 weeks’ gestation. Sequelae in survivors include cerebral palsy, intellectual disability, and seizure disorder.
Germinal matrix hemorrhage, microscopic
This transverse section of fetal brain shows a subependymal hemorrhage (►) arising within the darker blue, very cellular and metabolically active subependymal germinal matrix region. Hemorrhage extends into the ventricular space. This subependymal region has a high proliferation of neuroblasts that migrate into the cerebral parenchyma by 20 weeks’ gestation, then glioblasts proliferate, differentiate, and migrate until 32 weeks. This hemorrhage can rupture into the adjacent lateral ventricle to produce intraventricular hemorrhage and obstructive hydrocephalus, a feared complication of prematurity.

Intraventricular hemorrhage, gross
This coronal section of a newborn brain shows a very large dark subependymal hemorrhage extending into and dilating the ventricular system. Intraventricular hemorrhage (♦) can be severe, as shown here with blood filling and distending all the lateral ventricles, extending into brain parenchyma, and extending down the third ventricle and out the foramina into the subarachnoid space of brainstem. The prognosis with this extent of hemorrhage is grim. If the infant survives, resolution of the hemorrhage with scarring can lead to obstructive hydrocephalus.

Leukomalacia, gross
This child’s brain shows severe leukomalacia in which the white matter has become cystic and markedly shrunken (∗). The corpus callosum (▲) is only a thin band of tissue, and there is marked ex vacuo ventricular dilation (■) from loss of the hemispheric parenchyma. The overlying gray matter (▼) appears better preserved, although there is tissue loss in the neocortex as well. Affected patients usually experience an anoxic insult around the time of birth, or congenital infection, and become severely impaired neurologically. Periventricular leukomalacia may be marked by radiographic appearance of bright dystrophic calcifications in addition to the necrosis.

Ulegyria, gross
In this brain of a child, there is extensive loss of the cortical gray matter (▲) at the depths of these sulci with relative sparing of apical cortical portions, producing the mushroom-like appearance of gyri also observed with MR imaging. The thin gyral portions eventually become gliotic. Ulegyria is usually the result of an anoxic-ischemic event at or around the time of birth. The injury is most pronounced at the less perfused depths of the sulci, particularly in parasagittal watershed regions. Surviving children may have intellectual disability, various neurologic deficits in the category of cerebral palsy, and seizure disorders.

Status marmoratus, gross
This coronal section of brain through the basal ganglia with caudate and putamen shows an increased irregular white color (♦) of the basal ganglia. This is status marmoratus, or “marbled state,” owing to anoxia, which causes malfunction of the myelinating cells, the oligodendroglia, leading to abnormal myelination and the abnormal white areas seen here. Thalamus may also be affected. There is also neuronal loss and gliosis, which adds to the increased white color. Affected persons can have severe extrapyramidal movement problems, such as choreoathetosis.

Skull fracture, gross
Blunt force trauma to the head can lead to skull fracture. The right orbital plate at the base of this skull at autopsy shows multiple fractures (arrow) in an older patient who fell backward. The force of the blow was transmitted forward, resulting in a contrecoup injury pattern. Such basilar skull fractures may also occur with a blow to the side of the stationary head (coup injury pattern). A basal skull fracture may be suspected in a patient with a periorbital hematoma (dark discoloration of upper eyelids), blood collecting in mastoids, or cerebrospinal fluid rhinorrhea or otorrhea.

Skull fracture, radiograph
After a fall, this patient incurred a linear skull fracture, seen here as the long dark line (▲) in this lateral skull x-ray. The fainter branching gray lines represent the normal cranial vascular pattern outlined along the inner skull surface. If there is a direct blow to a stationary head, a coup injury with contusion to underlying brain can occur, even though the fracture is nondisplaced. In the absence of gross or imaging abnormalities to the brain, a more subtle concussion, defined by clinical findings, may have occurred.

Skull fracture, CT image
Head CT scan in “bone window” shows a skull fracture (►) on the right with diastasis of the sutures. However, this is not a depressed skull fracture, and it is not displaced; a displaced fracture results in skull bone extending into the cranial cavity for a distance greater than the skull thickness. A fracture in this location could result from a coup injury because of a direct blow to that portion of skull. Note the marked contusion with overlying soft-tissue swelling (♦) in the scalp, and a posterior small overlying skin laceration has been closed with a bright staple.

Cerebral contusions, gross
A coronal section through the frontal lobes reveals extensive recent contusions (bruises) with multiple superficial gyral hemorrhages (▲), most pronounced at the crests of the gyri, with relative sparing of cortex in the deeper sulci. More severe lesions could have extension of hemorrhage to underlying white matter. This contrecoup injury resulted from a fall backward, striking the occiput, with the force transmitted anteriorly to produce these contusions. In contrast, a coup type of brain injury occurs with a direct blow to the head and force delivered to the region of the brain adjacent to the site of impact. There can be subarachnoid hemorrhage and edema in the region of contusion, producing a local mass effect.

Cerebral contusions, CT image
The brighter areas (▲) of attenuation seen here within the cerebral parenchyma represent subfrontal contusions resulting from a contrecoup injury sustained in a fall backward. There is more hemorrhage and edema on the right, with a midline shift to the left and narrowing of ventricles. There was also subdural hemorrhage drained through a burr hole marked by the starburst artifact, with overlying four bright sutures as seen on the right.

Cerebral contusions, gross
The inferior surfaces of these frontal lobes and the right inferior temporal lobe tip display old hemosiderin-stained contusions (▲). They are slightly depressed from removal of necrotic cortex by macrophages and subsequent gliosis. These old lesions have been called plaques jaunes because of the yellow to brown discoloration from accumulation of hemosiderin derived from breakdown of blood in these superficial cortical hemorrhages. Persons with such contusions may develop a focal or partial seizure disorder years after the accident. There may be loss of smell (anosmia) if the olfactory bulbs and/or tracts are involved.
Diffuse axonal injury, microscopic
This silver stain of the centrum semiovale shows dark axonal retraction balls (▼) within white matter. These retraction balls can be formed after shearing force injuries. Such injuries can occur with rotational forces (angular acceleration or deceleration) or with violent shaking, as in “shaken infant” syndrome, or in individuals ejected from motor vehicles at high speed. The axons are stretched and broken at nodes of Ranvier, then undergo retraction, causing the axoplasm to compress into an enlarged ball. Such involved axonal fibers eventually degenerate. Focal hemorrhages may accompany these lesions. Half of patients in coma after head trauma may have these lesions.
Epidural hematoma, gross
Blunt force head trauma causing a tear in a meningeal artery leads to a collection of blood (♦) in the epidural space. This acute arterial bleeding occurs between the dura and the skull and quickly leads to hematoma formation, seen here at autopsy on the right from middle meningeal arterial bleeding. There is often a skull fracture accompanying this lesion. Because the arterial bleeding is brisk, these patients may have only a short lucid interval after the injury but quickly lapse into coma because of the brain compression by the expanding hematoma. If not emergently evacuated, the expanding mass of blood leads to herniation and death.
Epidural hematoma, CT image
Note the large right epidural hematoma with a lens-shaped outline (+), as the smooth dura becomes indented against the underlying cortex on the right lateral aspect of the cerebrum. The epidural hematoma is confined within an area bounded by cranial sutures where the dura is firmly adherent to the skull. This acute blood collection appears bright with CT scan. Note the mass effect with effacement of the lateral ventricles and the shift of midline to the left. In this case, the patient fell from a height and struck the right side of his head, severing the middle meningeal artery. This epidural hematoma formed acutely within hours.
Subdural hematoma, gross, and bridging veins, gross
The large subdural hematoma (♦) in the left panel overlies the left frontoparietal region. A subdural hematoma forms after head trauma that severs bridging veins from dura to brain, shown in the right panel, where the dura has been reflected to reveal the normal appearance of the bridging veins (◄) that extend across subdural space to the superior aspect of the cerebral hemispheres. Elderly patients and very young patients are at greater risk because their cerebral veins are more vulnerable to injury. The bleeding is venous, so blood collects over hours to weeks, with variable onset of symptoms. The blood collecting beneath the dura can be seen to cross the region of cranial sutures and interdigitate with underlying brain cortex.
Subdural hematomas, CT image
In the left panel a large left subdural hematoma (+) with left-to-right shift (◄) and ventricular narrowing interdigitates with the adjacent gyri and sulci but compresses the brain. In the right panel, bilateral subdural hematomas can be seen, the right one (+) greater in size than the left (×). There are irregular bright areas within these subdurals, indicating that the hemorrhage was relatively recent, but is not completely bright, so some organization of the hematoma has begun. Clot lysis generally occurs 1 week after hematoma formation, with appearance of granulation tissue, including fibroblastic proliferation from the dura, over the next week, and formation of a neomembrane of connective tissue within 1 to 3 months after the original injury. Symptoms of subdural hematoma have a variable onset from hours to weeks, depending on the amount of bleeding. Rebleeding from delicate vessels within the granulation tissue is common, leading to chronic subdural hematoma.
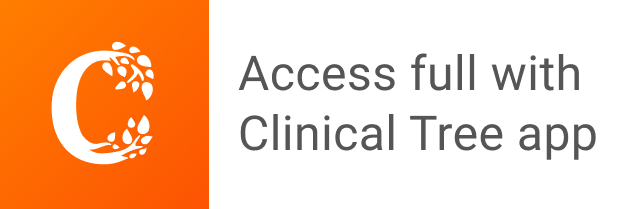