OBJECTIVES
After reading this chapter, you should be able to:
Describe the morphology of a typical nephron and its blood supply.
Define autoregulation and list the major theories advanced to explain autoregulation in the kidneys.
Define glomerular filtration rate, describe how it can be measured, and list the major factors affecting it.
Outline tubular handling of Na+ and water.
Discuss tubular reabsorption and secretion of glucose and K+.
Describe how the countercurrent mechanism in the kidney operates to produce hypertonic or hypotonic urine.
List the major classes of diuretics; understand how each operates to increase urine flow.
Describe the voiding reflex and draw a cystometrogram.
FUNCTIONAL ANATOMY
Each individual renal tubule and its glomerulus is a unit (nephron). The size of the kidneys between species varies, as does the number of nephrons they contain. Each human kidney has approximately 1 million nephrons. The specific structures of the nephron are shown in diagrammatic manner in Figure 37–1.
The glomerulus, which is about 200 μm in diameter, is formed by the invagination of a tuft of capillaries into the dilated, blind end of the nephron (Bowman’s capsule). The capillaries are supplied by an afferent arteriole and drained by the efferent arteriole (Figure 37–2), and it is from the glomerulus that the filtrate is formed. The diameter of the afferent arteriole is larger than the efferent arteriole. Two cellular layers separate the blood from the glomerular filtrate in Bowman’s capsule: the capillary endothelium and the specialized epithelium of the capsule. The endothelium of the glomerular capillaries is fenestrated, with pores that are 70–90 nm in diameter. The endothelium of the glomerular capillaries is completely surrounded by the glomerular basement membrane along with specialized cells called podocytes. Podocytes have numerous pseudopodia that interdigitate (Figure 37–2) to form filtration slits along the capillary wall. The slits are approximately 25 nm wide, and each is closed by a thin membrane. The glomerular basement membrane, the basal lamina, does not contain visible gaps or pores. Stellate cells called mesangial cells are located between the basal lamina and the endothelium. They are similar to cells called pericytes, which are found in the walls of capillaries elsewhere in the body. Mesangial cells are especially common between two neighboring capillaries, and in these locations the basal membrane forms a sheath shared by both capillaries (Figure 37–2). The mesangial cells are contractile and play a role in the regulation of glomerular filtration. Mesangial cells secrete the extracellular matrix, take up immune complexes, and are involved in the progression of glomerular disease.
FIGURE 37–2
Structural details of glomerulus. A) Section through vascular pole, showing capillary loops. B) Relation of mesangial cells and podocytes to glomerular capillaries. C) Detail of the way podocytes form filtration slits on the basal lamina, and the relation of the lamina to the capillary endothelium. D) Enlargement of the rectangle in C to show the podocyte processes. The fuzzy material on their surfaces is glomerular polyanion.
Functionally, the glomerular membrane permits the free passage of neutral substances up to 4 nm in diameter and almost totally excludes those with diameters greater than 8 nm. However, the charge on molecules as well as their diameters affects their passage into Bowman’s capsule. The total area of glomerular capillary endothelium across which filtration occurs in humans is about 0.8 m2.
The general features of the cells that make up the walls of the tubules are shown in Figure 37–1; however, there are cell subtypes in all segments, and the anatomic differences between them correlate with differences in function.
The human proximal convoluted tubule is about 15 mm long and 55 μm in diameter. Its wall is made up of a single layer of cells that interdigitate with one another and are united by apical tight junctions. Between the cells are extensions of the extracellular space called the lateral intercellular spaces. The luminal edges of the cells have a striated brush border due to the presence of many microvilli.
The convoluted proximal tubule straightens and the next portion of each nephron is the loop of Henle. The descending portion of the loop and the proximal portion of the ascending limb are made up of thin, permeable cells. On the other hand, the thick portion of the ascending limb (Figure 37–1) is made up of thick cells containing many mitochondria. The nephrons with glomeruli in the outer portions of the renal cortex have short loops of Henle (cortical nephrons), whereas those with glomeruli in the juxtamedullary region of the cortex (juxtamedullary nephrons) have long loops extending down into the medullary pyramids. In humans, only 15% of the nephrons have long loops.
The thick end of the ascending limb of the loop of Henle reaches the glomerulus of the nephron from which the tubule arose and nestles between its afferent and efferent arterioles. Specialized cells at the end form the macula densa, which is close to the efferent and particularly the afferent arteriole (Figure 37–2). The macula, the neighboring lacis cells, and the renin-secreting granular cells in the afferent arteriole form the juxtaglomerular apparatus (see Figure 38–8).
The distal convoluted tubule, which starts at the macula densa, is about 5 mm long. Its epithelium is lower than that of the proximal tubule, and although a few microvilli are present, there is no distinct brush border. The distal tubules coalesce to form collecting ducts that are about 20 mm long and pass through the renal cortex and medulla to empty into the pelvis of the kidney at the apexes of the medullary pyramids. The epithelium of the collecting ducts is made up of principal cells (P cells) and intercalated cells (I cells). The P cells, which predominate, are relatively tall and have few organelles. They are involved in Na+ reabsorption and vasopressin-stimulated water reabsorption. The I cells, which are present in smaller numbers and are also found in the distal tubules, have more microvilli, cytoplasmic vesicles, and mitochondria. They are concerned with acid secretion and HCO3– transport. The total length of the nephrons, including the collecting ducts, ranges from 45 to 65 mm.
Cells in the kidneys that appear to have a secretory function include not only the granular cells in the juxtaglomerular apparatus but also some of the cells in the interstitial tissue of the medulla. These cells are called renal medullary interstitial cells (RMICs) and are specialized fibroblast-like cells. They contain lipid droplets and are a major site of cyclooxygenase 2 (COX-2) and prostaglandin synthase (PGES) expression. PGE2 is the major prostanoid synthesized in the kidney and is an important paracrine regulator of salt and water homeostasis. PGE2 is secreted by the RMICs, by the macula densa, and by cells in the collecting ducts; prostacyclin (PGI2) and other prostaglandins are secreted by the arterioles and glomeruli.
The renal circulation is diagrammed in Figure 37–3. The afferent arterioles are short, straight branches of the interlobular arteries. Each divides into multiple capillary branches to form the tuft of vessels in the glomerulus. The capillaries coalesce to form the efferent arteriole, which in turn breaks up into capillaries that supply the tubules (peritubular capillaries) before draining into the interlobular veins. The arterial segments between glomeruli and tubules are thus technically a portal system, and the glomerular capillaries are the only capillaries in the body that drain into arterioles. However, there is relatively little smooth muscle in the efferent arterioles.
FIGURE 37–3
Renal circulation. Interlobar arteries divide into arcuate arteries, which give off interlobular arteries in the cortex. The interlobular arteries provide an afferent arteriole to each glomerulus. The efferent arteriole from each glomerulus breaks up into capillaries that supply blood to the renal tubules. Venous blood enters interlobular veins, which in turn flow via arcuate veins to the interlobar veins. (Modified with permission from Boron WF, Boulpaep EL: Medical Physiology. Saunders, 2009.)
The capillaries draining the tubules of the cortical nephrons form a peritubular network, whereas the efferent arterioles from the juxtamedullary glomeruli drain not only into a peritubular network, but also into vessels that form hairpin loops (the vasa recta). These loops dip into the medullary pyramids alongside the loops of Henle (Figure 37–3). The descending vasa recta have a nonfenestrated endothelium that contains a facilitated transporter for urea, and the ascending vasa recta have a fenestrated endothelium, consistent with their function in conserving solutes.
The efferent arteriole from each glomerulus breaks up into capillaries that supply a number of different nephrons. Thus, the tubule of each nephron does not necessarily receive blood solely from the efferent arteriole of the same nephron. In humans, the total surface of the renal capillaries is approximately equal to the total surface area of the tubules, both being about 12 m2. The volume of blood in the renal capillaries at any given time is 30–40 mL.
The kidneys have an abundant lymphatic supply that drains via the thoracic duct into the venous circulation in the thorax.
The renal capsule is thin but tough. If the kidney becomes edematous, the capsule limits the swelling, and the tissue pressure (renal interstitial pressure) rises. This decreases the glomerular filtration rate (GFR) and is claimed to enhance and prolong anuria in acute kidney injury (AKI).
The renal nerves travel along the renal blood vessels as they enter the kidney. They contain many postganglionic sympathetic efferent fibers and a few afferent fibers. There also appears to be a cholinergic innervation via the vagus nerve, but its function is uncertain. The sympathetic preganglionic innervation comes primarily from the lower thoracic and upper lumbar segments of the spinal cord, and the cell bodies of the postganglionic neurons are in the sympathetic ganglion chain, in the superior mesenteric ganglion, and along the renal artery. The sympathetic fibers are distributed primarily to the afferent and efferent arterioles, the proximal and distal tubules, and the juxtaglomerular apparatus (see Chapter 38). In addition, there is a dense noradrenergic innervation of the thick ascending limb of the loop of Henle.
Nociceptive afferents that mediate pain in kidney disease parallel the sympathetic efferents and enter the spinal cord in the thoracic and upper lumbar dorsal roots. Other renal afferents presumably mediate a renorenal reflex by which an increase in ureteral pressure in one kidney leads to a decrease in efferent nerve activity to the contralateral kidney. This decrease permits an increase in its excretion of Na+ and water.
RENAL CIRCULATION
In a resting adult, the kidneys receive 1.2–1.3 L of blood per minute, or just under 25% of the cardiac output. Renal blood flow can be measured with electromagnetic or other types of flow meters, or it can be determined by applying the Fick principle (see Chapter 30) to the kidney; that is, by measuring the amount of a given substance taken up per unit of time and dividing this value by the arteriovenous difference for the substance across the kidney. Because the kidney filters plasma, the renal plasma flow (RPF) equals the amount of a substance excreted per unit of time divided by the renal arteriovenous difference as long as the amount in the red cells is unaltered during passage through the kidney. Any excreted substance can be used if its concentration in arterial and renal venous plasma can be measured and if it is not metabolized, stored, or produced by the kidney and does not itself affect blood flow.
RPF can be measured by infusing p-aminohippuric acid (PAH) and determining its urine and plasma concentrations. PAH is filtered by the glomeruli and secreted by the tubular cells, so that its extraction ratio (arterial concentration minus renal venous concentration divided by arterial concentration) is high. For example, when PAH is infused at low doses, 90% of the PAH in arterial blood is removed in a single circulation through the kidney. It has therefore become commonplace to calculate the “RPF” by dividing the amount of PAH in the urine by the plasma PAH level, ignoring the level in renal venous blood. Peripheral venous plasma can be used because its PAH concentration is essentially identical to that in the arterial plasma reaching the kidney. The value obtained should be called the effective renal plasma flow (ERPF) to indicate that the level in renal venous plasma was not measured. In humans, ERPF averages about 625 mL/min.
Example:
Concentration of PAH in urine (UPAH): 14 mg/mL
Urine flow : 0.9 mL/min
Concentration of PAH in plasma (PPAH): 0.02 mg/mL
It should be noted that the ERPF determined in this way is the clearance of PAH. The concept of clearance is discussed in detail below.
ERPF can be converted to actual renal plasma flow (RPF):
Average PAH extraction ratio: 0.9
From the renal plasma flow, the renal blood flow can be calculated by dividing by 1 minus the hematocrit:
Hematocrit (Hct): 45%
The pressure in the glomerular capillaries has been measured directly in rats and has been found to be considerably lower than predicted on the basis of indirect measurements. When the mean systemic arterial pressure is 100 mm Hg, the glomerular capillary pressure is about 45 mm Hg. The pressure drop across the glomerulus is only 1–3 mm Hg, but a further drop occurs in the efferent arteriole so that the pressure in the peritubular capillaries is about 8 mm Hg. The pressure in the renal vein is about 4 mm Hg. Pressure gradients are similar in squirrel monkeys and presumably in humans, with a glomerular capillary pressure that is about 40% of systemic arterial pressure.
Norepinephrine (noradrenaline) constricts the renal vessels, with the greatest effect of injected norepinephrine being exerted on the interlobular arteries and the afferent arterioles. Dopamine is made in the kidney and causes renal vasodilation and natriuresis. Angiotensin II exerts a constrictor effect on both the afferent and efferent arterioles. Prostaglandins increase blood flow in the renal cortex and decrease blood flow in the renal medulla. Acetylcholine also produces renal vasodilation. A high-protein diet raises glomerular capillary pressure and increases renal blood flow.
Stimulation of the renal nerves increases renin secretion by a direct action of released norepinephrine on β1-adrenergic receptors on the juxtaglomerular cells (see Chapter 38), and it increases Na+ reabsorption, probably by a direct action of norepinephrine on renal tubular cells. The proximal and distal tubules and the thick ascending limb of the loop of Henle are richly innervated. When the renal nerves are stimulated to increasing extents in experimental animals, the first response is an increase in the sensitivity of the granular cells in the juxtaglomerular apparatus (Table 37–1), followed by increased renin secretion, then increased Na+ reabsorption, and finally, at the highest threshold, renal vasoconstriction with decreased glomerular filtration and renal blood flow. It is still unsettled whether the effect on Na+ reabsorption is mediated via α- or β-adrenergic receptors, and it may be mediated by both. The physiologic role of the renal nerves in Na+ homeostasis is also unsettled, in part because most renal functions appear to be normal in patients with transplanted kidneys, and it takes some time for transplanted kidneys to acquire a functional innervation.
Renal Nerve Stimulation Frequency (Hz) | RSR | UNaV | GFR | RBF |
---|---|---|---|---|
0.25 | No effect on basal values; augments RSR mediated by nonneural stimuli | 0 | 0 | 0 |
0.50 | Increased without changing UNaV, GFR, or RBF | 0 | 0 | 0 |
1.0 | Increased with decreased UNaV, without changing GFR or RBF | ↓ | 0 | 0 |
2.50 | Increased with decreased UNaV, GFR, and RBF | ↓ | ↓ | ↓ |
Strong stimulation of the sympathetic noradrenergic nerves to the kidneys causes a marked decrease in renal blood flow. This effect is mediated by α1-adrenergic receptors and to a lesser extent by postsynaptic α2-adrenergic receptors. Some tonic discharge takes place in the renal nerves at rest in animals and humans. When systemic blood pressure falls, the vasoconstrictor response produced by decreased discharge in the baroreceptor nerves includes renal vasoconstriction. Renal blood flow is decreased during exercise and, to a lesser extent, on rising from the supine position.
When the kidney is perfused at moderate pressures (90–220 mm Hg in the dog), the renal vascular resistance varies with the pressure so that renal blood flow is relatively constant (Figure 37–4). Autoregulation of this type occurs in other organs, and several factors contribute to it (see Chapter 32). Renal autoregulation is present in denervated and in isolated, perfused kidneys but is prevented by the administration of drugs that paralyze vascular smooth muscle. It is probably produced in part by a direct contractile response to stretch of the smooth muscle of the afferent arteriole. NO may also be involved. At low perfusion pressures, angiotensin II also appears to play a role by constricting the efferent arterioles, thus maintaining the GFR. This is believed to be the explanation of the renal failure that sometimes develops in patients with poor renal perfusion who are treated with drugs that inhibit angiotensin-converting enzyme.
The main function of the renal cortex is filtration of large volumes of blood through the glomeruli, so it is not surprising that the renal cortical blood flow is relatively great and little oxygen is extracted from the blood. Cortical blood flow is about 5 mL/g of kidney tissue/min (compared with 0.5 mL/g/min in the brain), and the arteriovenous oxygen difference for the whole kidney is only 14 mL/L of blood, compared with 62 mL/L for the brain and 114 mL/L for the heart (see Table 33–1). The Po2 of the cortex is about 50 mm Hg. On the other hand, maintenance of the osmotic gradient in the medulla requires a relatively low blood flow. It is not surprising, therefore, that the blood flow is about 2.5 mL/g/min in the outer medulla and 0.6 mL/g/min in the inner medulla. However, metabolic work is being done, particularly to reabsorb Na+ in the thick ascending limb of Henle, so relatively large amounts of O2 are extracted from the blood in the medulla. The Po2 of the medulla is about 15 mm Hg. This makes the medulla vulnerable to hypoxia if flow is reduced further. NO, prostaglandins, and many cardiovascular peptides in this region function in a paracrine manner to maintain the balance between low blood flow and metabolic needs.
GLOMERULAR FILTRATION
Glomerular filtration rate (GFR) is the amount of plasma ultrafiltrate formed each minute and can be measured in intact experimental animals and humans by measuring the plasma level of a substance and the amount of that substance that is excreted. A substance to be used to measure GFR must be freely filtered through the glomeruli and must be neither secreted nor reabsorbed by the tubules.
In addition to the requirement that it be freely filtered and neither reabsorbed nor secreted in the tubules, a substance suitable for measuring the GFR should be nontoxic and not metabolized by the body. Inulin, a polymer of fructose with a molecular weight of 5200, meets these criteria in humans and most animals and can be used to measure GFR.
Renal plasma clearance is the volume of plasma from which a substance is completely removed by the kidney in a given amount of time (usually minutes). The amount of that substance that appears in the urine per unit of time is the result of the renal filtering of a certain number of milliliters of plasma that contained this amount. GFR and clearance are measured in mL/min.
Therefore, if the substance is designated by the letter X, the GFR is equal to the concentration of X in urine (UX) times the urine flow per unit of time divided by the arterial plasma level of X (PX), or
/PX. This value is called the clearance of X (CX).
In practice, a loading dose of inulin is administered intravenously, followed by a sustaining infusion to keep the arterial plasma level constant. After the inulin has equilibrated with body fluids, an accurately timed urine specimen is collected and a plasma sample obtained halfway through the collection. Plasma and urinary inulin concentrations are determined and the clearance is calculated:
Clearance of creatinine (CCr) can also be used to determine GFR; however, some creatinine is secreted by the tubules, thus the clearance of creatinine will be slightly higher than inulin. In spite of this, the clearance of endogenous creatinine is a reasonable estimate of GFR as the values agree quite well with the GFR values measured with inulin (see Table 37–2). More common though is the use of PCr values as an index of renal function (normal = 1 mg/dL).
The GFR in a healthy adult of average size is approximately 125 mL/min. Its magnitude correlates fairly well with surface area, but values in women are 10% lower than those in men even after correction for surface area. A rate of 125 mL/min is 7.5 L/h, or 180 L/d, whereas the normal urine volume is about 1 L/d. Thus, 99% or more of the filtrate is normally reabsorbed. At the rate of 125 mL/min, in 1 day the kidneys filter an amount of fluid equal to four times the total body water, 15 times the ECF volume, and 60 times the plasma volume.
The factors governing filtration across the glomerular capillaries are the same as those governing filtration across all other capillaries (see Chapter 31), that is, the size of the capillary bed, the permeability of the capillaries, and the hydrostatic and osmotic pressure gradients across the capillary wall. For each nephron:
where Kf, the glomerular ultrafiltration coefficient, is the product of the glomerular capillary wall hydraulic conductivity (ie, its permeability) and the effective filtration surface area. PGC is the mean hydrostatic pressure in the glomerular capillaries, PT the mean hydrostatic pressure in the tubule (Bowman’s space), πGC the oncotic pressure of the plasma in the glomerular capillaries, and πT the oncotic pressure of the filtrate in the tubule (Bowman’s space).
The permeability of the glomerular capillaries is about 50 times that of the capillaries in skeletal muscle. Neutral substances with effective molecular diameters of less than 4 nm are freely filtered, and the filtration of neutral substances with diameters of more than 8 nm approaches zero. Between these values, filtration is inversely proportional to diameter. However, sialoproteins in the glomerular capillary wall are negatively charged, and studies with negatively and positively charged dextrans indicate that the negative charges repel negatively charged substances in blood, with the result that filtration of anionic substances 4 nm in diameter is less than half that of neutral substances of the same size. This probably explains why albumin, with an effective molecular diameter of approximately 7 nm, normally has a glomerular concentration only 0.2% of its plasma concentration rather than the higher concentration that would be expected on the basis of diameter alone; circulating albumin is negatively charged. Conversely, filtration of cationic substances is greater than that of neutral substances.
The amount of protein in the urine is normally less than 100 mg/day, and most of this is not filtered but comes from shed tubular cells. The presence of significant amounts of albumin in the urine is called albuminuria. In nephritis, the negative charges in the glomerular wall are dissipated, and albuminuria can occur for this reason without an increase in the size of the “pores” in the membrane.
Kf can be altered by the mesangial cells, with contraction of these cells producing a decrease in Kf that is largely due to a reduction in the area available for filtration. Contraction of points where the capillary loops bifurcate probably shifts flow away from some of the loops, and elsewhere, contracted mesangial cells distort and encroach on the capillary lumen. Agents that have been shown to affect the mesangial cells are listed in Table 37–3. Angiotensin II is an important regulator of mesangial contraction, and there are angiotensin II receptors in the glomeruli. In addition, some evidence suggests that mesangial cells make renin.
The pressure in the glomerular capillaries is higher than that in other capillary beds because the afferent arterioles are short, straight branches of the interlobular arteries. Furthermore, the vessels “downstream” from the glomeruli, the efferent arterioles, have a relatively high resistance. The capillary hydrostatic pressure is opposed by the hydrostatic pressure in Bowman’s capsule. It is also opposed by the oncotic pressure gradient across the glomerular capillaries (πGC – πT). πT is normally negligible, and the gradient is essentially equal to the oncotic pressure of the plasma proteins.
The actual pressures in one strain of rats are shown in Figure 37–5. The net filtration pressure (PUF) is 15 mm Hg at the afferent end of the glomerular capillaries, but it falls to zero—that is, filtration equilibrium is reached—proximal to the efferent end of the glomerular capillaries. This is because fluid leaves the plasma and the oncotic pressure rises as blood passes through the glomerular capillaries. The calculated change in Δπ along an idealized glomerular capillary is also shown in Figure 37–5. It is apparent that portions of the glomerular capillaries do not normally contribute to the formation of the glomerular ultrafiltrate; that is, exchange across the glomerular capillaries is flow-limited rather than diffusion-limited. It is also apparent that a decrease in the rate of rise of the Δ curve produced by an increase in RPF would increase filtration because it would increase the distance along the capillary in which filtration was taking place.
FIGURE 37–5
Hydrostatic pressure (PGC) and osmotic pressure (πGC) in a glomerular capillary in the rat. PT, pressure in Bowman’s capsule; PUF, net filtration pressure. πT is normally negligible, so Δπ = πGC. ΔP = PGC – PT. (Reproduced with permission from Mercer PF, Maddox DA, Brenner BM: Current concepts of sodium chloride and water transport by the mammalian nephron. West J Med 1974; Jan; 120(1):33–45.)
There is considerable species variation in whether filtration equilibrium is reached, and some uncertainties are inherent in the measurement of Kf. It is uncertain whether filtration equilibrium is reached in humans.
Variations in the factors discussed in the preceding paragraphs and listed in Table 37–4 have predictable effects on the GFR. Changes in renal vascular resistance as a result of autoregulation tend to stabilize filtration pressure, but when the mean systemic arterial pressure drops below the autoregulatory range (Figure 37–4), GFR drops sharply. The GFR tends to be maintained when efferent arteriolar constriction is greater than afferent constriction, but either type of constriction decreases blood flow to the tubules.
Changes in renal blood flow |
Changes in glomerular capillary hydrostatic pressure |
Changes in systemic blood pressure |
Afferent or efferent arteriolar constriction |
Changes in hydrostatic pressure in Bowman’s capsule |
Ureteral obstruction |
Edema of kidney inside tight renal capsule |
Changes in concentration of plasma proteins: dehydration, hypoproteinemia, etc (minor factors) |
Changes in Kf |
Changes in glomerular capillary permeability |
Changes in effective filtration surface area |
The ratio of the GFR to the RPF, the filtration fraction, is normally 0.16–0.20. The GFR varies less than the RPF. When there is a fall in systemic blood pressure, the GFR falls less than the RPF because of efferent arteriolar constriction, and consequently the filtration fraction rises.
TUBULAR FUNCTION
The amount of any substance (X) that is filtered is the product of the GFR and the plasma level of the substance (ClnPX). The tubular cells may add more of the substance to the filtrate (tubular secretion), may remove some or all of the substance from the filtrate (tubular reabsorption), or may do both. The amount of the substance excreted per unit of () time equals the amount filtered plus the net amount transferred by the tubules. This latter quantity is conveniently indicated by the symbol TX (Figure 37–6). The clearance of the substance equals the GFR if there is no net tubular secretion or reabsorption, exceeds the GFR if there is net tubular secretion, and is less than the GFR if there is net tubular reabsorption.
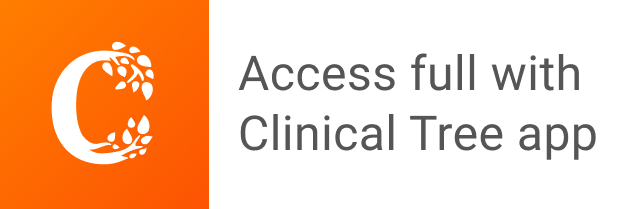