OBJECTIVES
After studying this chapter, you should be able to:
Locate the pre-Bötzinger complex and describe its role in producing spontaneous respiration.
Identify the location and probable functions of the dorsal and ventral groups of respiratory neurons, the pneumotaxic center, and the apneustic center in the brainstem.
List the specific respiratory functions of the vagus nerves and the respiratory receptors in the carotid body, the aortic body, and the ventral surface of the medulla oblongata.
Describe and explain the ventilatory responses to increased CO2 concentrations in the inspired air.
Describe and explain the ventilatory responses to decreased O2 concentrations in the inspired air.
Describe the effects of each of the main nonchemical factors that influence respiration.
Describe the effects of exercise on ventilation and O2 exchange in the tissues.
Define periodic breathing and explain its occurrence in various disease states.
INTRODUCTION
Spontaneous respiration is produced by rhythmic discharge of motor neurons that innervate the respiratory muscles. This discharge is totally dependent on nerve impulses from the brain; breathing stops if the spinal cord is transected above the origin of the phrenic nerves. The rhythmic discharges from the brain that produce spontaneous respiration are regulated by alterations in arterial Po2, Pco2, and H+ concentration, and this chemical control of breathing is supplemented by a number of nonchemical influences. The physiologic bases for these phenomena are discussed in this chapter.
NEURAL CONTROL OF BREATHING
Two separate neural mechanisms regulate respiration. One is responsible for voluntary control and the other for automatic control. The voluntary system is located in the cerebral cortex and sends impulses to the respiratory motor neurons via the corticospinal tracts. The automatic system is driven by a group of pacemaker cells in the medulla. Impulses from these cells activate motor neurons in the cervical and thoracic spinal cord that innervate inspiratory muscles. Those in the cervical cord activate the diaphragm via the phrenic nerves, and those in the thoracic spinal cord activate the external intercostal muscles. However, the impulses also reach the innervation of the internal intercostal muscles and other expiratory muscles.
The motor neurons to the expiratory muscles are inhibited when those supplying the inspiratory muscles are active, and vice versa. Although spinal reflexes contribute to this reciprocal innervation, it is due primarily to activity in descending pathways. Impulses in these descending pathways excite agonists and inhibit antagonists. The one exception to the reciprocal inhibition is a small amount of activity in phrenic axons for a short period after inspiration. The function of this postinspiratory output appears to be to brake the lung’s elastic recoil and make respiration smooth.
The main components of the respiratory control pattern generator responsible for automatic respiration are located in the medulla. Rhythmic respiration is initiated by a small group of synaptically coupled pacemaker cells in the pre-Bötzinger complex (pre-BÖTC) on either side of the medulla between the nucleus ambiguus and the lateral reticular nucleus (Figure 36–1). These neurons discharge rhythmically, and they produce rhythmic discharges in phrenic motor neurons that are abolished by sections between the pre-BÖTC complex and these motor neurons. They also contact the hypoglossal nuclei, and the tongue is involved in the regulation of airway resistance.
FIGURE 36–1
Pacemaker cells in the pre-Bötzinger complex (pre-BÖTC). Top: Anatomic diagram of the pre-BÖTC from a neonatal rat. Bottom: Sample rhythmic discharge tracing of neurons in the pre-BÖTC complex from a brain slice of a neonatal rat. IO, inferior olive; LRN, lateral reticular nucleus; NA, nucleus ambiguus; XII, nucleus of 12th cranial nerve; 5SP, spinal nucleus of trigeminal nerve. (Modified with permission from Feldman JC, Gray PA: Sighs and gasps in a dish, Nat Neurosci 2000; June; 3(6):531–532.)
Neurons in the pre-BÖTC complex discharge rhythmically in brain slice preparations in vitro, and if the slices become hypoxic, discharge changes to one associated with gasping. Addition of cadmium to the slices causes occasional sigh-like discharge patterns. There are NK1 receptors and μ-opioid receptors on these neurons, and, in vivo, substance P stimulates and opioids inhibit respiration. Depression of respiration is a side effect that limits the use of opioids in the treatment of pain. However, it is now known that 5HT4 receptors are present in the pre-BÖTC complex and treatment with 5HT4 agonists blocks the inhibitory effect of opioids on respiration in experimental animals, without inhibiting their analgesic effect.
In addition, dorsal and ventral groups of respiratory neurons are present in the medulla (Figure 36–2). However, lesions of these neurons do not abolish respiratory activity, and they apparently project to the pre-BÖTC pacemaker neurons.
FIGURE 36–2
Respiratory neurons in the brainstem. Dorsal view of brainstem; cerebellum removed. The effects of various lesions and brainstem transections are shown; the spirometer tracings at the right indicate the depth and rate of breathing. If a lesion is introduced at D, breathing ceases. The effects of higher transections, with and without vagus nerves transection, are shown (see text for details). CP, middle cerebellar peduncle; DRG, dorsal group of respiratory neurons; IC, inferior colliculus; NPBL, nucleus parabrachialis (pneumotaxic center); VRG, ventral group of respiratory neurons; 4th vent, fourth ventricle. The roman numerals identify cranial nerves. (Modified with permission from Mitchell RA, Berger A: State of the art: Review of neural regulation of respiration. Am Rev Respir Dis 1975; Feb; 111(2):206–224.)
Although the rhythmic discharge of medullary neurons concerned with respiration is spontaneous, it is modified by neurons in the pons and afferents in the vagus from receptors in the airways and lungs. An area known as the pneumotaxic center in the medial parabrachial and Kölliker-Fuse nuclei of the dorsolateral pons contains neurons active during inspiration and neurons active during expiration. When this area is damaged, respiration becomes slower and tidal volume greater, and when the vagi are also cut in anesthetized animals, there are prolonged inspiratory spasms that resemble breath holding (apneusis; section B in Figure 36–2). The normal function of the pneumotaxic center is unknown, but it may play a role in switching between inspiration and expiration.
Stretching of the lungs during inspiration initiates impulses in afferent pulmonary vagal fibers. These impulses inhibit inspiratory discharge. This is why the depth of inspiration is increased after vagotomy (Figure 36–2) and apneusis develops if the vagi are cut after damage to the pneumotaxic center. Vagal feedback activity does not alter the rate of rise of the neural activity in respiratory motor neurons (Figure 36–3).
FIGURE 36–3
Afferent vagal fibers inhibit inspiratory discharge. Superimposed records of two breaths: A) with and B) without feedback vagal afferent activity from stretch receptors in the lungs. Note that the rate of rise in phrenic nerve activity to the diaphragm is unaffected but the discharge is prolonged in the absence of vagal input.
When the activity of the inspiratory neurons is increased in intact animals, the rate and the depth of breathing are increased. The depth of respiration is increased because the lungs are stretched to a greater degree before the amount of vagal and pneumotaxic center inhibitory activity is sufficient to overcome the more intense inspiratory neuron discharge. The respiratory rate is increased because the after-discharge in the vagal and possibly the pneumotaxic afferents to the medulla is rapidly overcome.
REGULATION OF RESPIRATORY ACTIVITY
A rise in the Pco2 or H+ concentration of arterial blood or a drop in its Po2 increases the level of respiratory neuron activity in the medulla, and changes in the opposite direction have a slight inhibitory effect. The effects of variations in blood chemistry on ventilation are mediated via respiratory chemoreceptors—the carotid and aortic bodies and collections of cells in the medulla and elsewhere that are sensitive to changes in the chemistry of the blood. They initiate impulses that stimulate the respiratory center. Superimposed on this basic chemical control of respiration, other afferents provide nonchemical controls that affect breathing in particular situations (Table 36–1).
Chemical control |
CO2 (via CSF and brain interstitial fluid H+ concentration) |
![]() |
Nonchemical control |
Vagal afferents from receptors in the airways and lungs |
Afferents from the pons, hypothalamus, and limbic system |
Afferents from proprioceptors |
Afferents from baroreceptors: arterial, atrial, ventricular, pulmonary |
CHEMICAL CONTROL OF BREATHING
The chemical regulatory mechanisms adjust ventilation in such a way that the alveolar Pco2 is normally held constant, the effects of excess H+ in the blood are combated, and the Po2 is raised when it falls to a potentially dangerous level. The respiratory minute volume is proportional to the metabolic rate, but the link between metabolism and ventilation is CO2, not O2. The receptors in the carotid and aortic bodies are stimulated by a rise in the Pco2 or H+ concentration of arterial blood or a decline in its Po2. After denervation of the carotid chemoreceptors, the response to a drop in Po2 is abolished; the predominant effect of hypoxia after denervation of the carotid bodies is a direct depression of the respiratory center. The response to changes in arterial blood H+ concentration in the pH 7.3–7.5 range is also abolished, although larger changes exert some effect. The response to changes in arterial Pco2, on the other hand, is affected only slightly; it is reduced no more than 30–35%.
There is a carotid body near the carotid bifurcation on each side, and there are usually two or more aortic bodies near the arch of the aorta (Figure 36–4). Each carotid and aortic body (glomus) contains islands of two types of cells, type I and type II cells, surrounded by fenestrated sinusoidal capillaries. The type I or glomus cells are closely associated with cuplike endings of the afferent nerves (Figure 36–5). The glomus cells resemble adrenal chromaffin cells and have dense-core granules containing catecholamines that are released upon exposure to hypoxia and cyanide. The cells are excited by hypoxia, and the principal transmitter appears to be dopamine, which excites the nerve endings by way of D2 receptors. The type II cells are glia-like, and each surrounds four to six type I cells. The function of type II cells is not fully defined.
FIGURE 36–5
Organization of the carotid body. Type I (glomus) cells contain catecholamines. When exposed to hypoxia, they release their catecholamines, which stimulate the cuplike endings of the carotid sinus nerve fibers in the glossopharyngeal nerve. The glia-like type II cells surround the type I cells and probably have a sustentacular function.
Outside the capsule of each body, the nerve fibers acquire a myelin sheath; however, they are only 2–5 μm in diameter and conduct at the relatively low rate of 7–12 m/s. Afferents from the carotid bodies ascend to the medulla via the carotid sinus and glossopharyngeal nerves, and fibers from the aortic bodies ascend in the vagi. Studies in which one carotid body has been isolated and perfused while recordings are being taken from its afferent nerve fibers show that there is a graded increase in impulse traffic in these afferent fibers as the Po2 of the perfusing blood is lowered (Figure 36–6) or the Pco2 is raised.
FIGURE 36–6
Effect of Pco2 on afferent nerve firing. The rate of discharge of a single afferent fiber from the carotid body (circles) is plotted at several Po2 values and fitted to a line. A sharp increase in firing rate is observed as Po2 falls below normal resting levels (ie, near 100 mm Hg). (Used with permission of S. Sampson.)
Type I glomus cells have O2-sensitive K+ channels, whose conductance is reduced in proportion to the degree of hypoxia to which they are exposed. This reduces the K+ efflux, depolarizing the cell and causing Ca2+ influx, primarily via L-type Ca2+ channels. The Ca2+ influx triggers action potentials and transmitter release, with consequent excitation of the afferent nerve endings. The smooth muscle of pulmonary arteries contains similar O2-sensitive K+ channels, which mediate the vasoconstriction caused by hypoxia. This is in contrast to systemic arteries, which contain adenosine triphosphate- (ATP-) dependent K+ channels that permit more K+ efflux with hypoxia and consequently cause vasodilation instead of vasoconstriction.
The blood flow in each 2 mg carotid body is about 0.04 mL/min, or 2000 mL/100 g of tissue/min compared with a blood flow of 54 mL or 420 mL per 100 g/min in the brain and kidneys, respectively. Because the blood flow per unit of tissue is so enormous, the O2 needs of the cells can be met largely by dissolved O2 alone. Therefore, the receptors are not stimulated in conditions such as anemia or carbon monoxide poisoning, in which the amount of dissolved O2 in the blood reaching the receptors is generally normal, even though the combined O2 in the blood is markedly decreased. The receptors are stimulated when the arterial Po2 is low or when, because of vascular stasis, the amount of O2 delivered to the receptors per unit time is decreased. Powerful stimulation is also produced by cyanide, which prevents O2 utilization at the tissue level. In sufficient doses, nicotine and lobeline activate the chemoreceptors. It has also been reported that infusion of K+ increases the discharge rate in chemoreceptor afferents, and because the plasma K+ level is increased during exercise, the increase may contribute to exercise-induced hyperpnea.
Because of their anatomic location, the aortic bodies have not been studied in as great detail as the carotid bodies. Their responses are probably similar but of lesser magnitude. In humans in whom both carotid bodies have been removed but the aortic bodies left intact, the responses are essentially the same as those following denervation of both carotid and aortic bodies in animals: little change in ventilation at rest, but the ventilatory response to hypoxia is lost and the ventilatory response to CO2 is reduced by 30%.
Neuroepithelial bodies composed of innervated clusters of amine-containing cells are found in the airways. These cells have an outward K+ current that is reduced by hypoxia, and this would be expected to produce depolarization. However, the function of these hypoxia-sensitive cells is uncertain because, as noted above, removal of the carotid bodies alone abolishes the respiratory response to hypoxia.
The chemoreceptors that mediate the hyperventilation produced by increases in arterial Pco2 after the carotid and aortic bodies are denervated are located in the medulla oblongata and consequently are called medullary chemoreceptors. They are separate from the dorsal and ventral respiratory neurons and are located on the ventral surface of the medulla (Figure 36–7). Recent evidence indicates that additional chemoreceptors are located in the vicinity of the solitary tract nuclei, the locus ceruleus, and the hypothalamus.
The chemoreceptors monitor the H+ concentration of cerebrospinal fluid (CSF), including the brain interstitial fluid. CO2 readily penetrates membranes, including the blood–brain barrier, whereas H+ and HCO3− penetrate slowly. The CO2 that enters the brain and CSF is promptly hydrated. The H2CO3 dissociates, so that the local H+ concentration rises. The H+ concentration in brain interstitial fluid parallels the arterial Pco2. Experimentally produced changes in the Pco2 of CSF have minor, variable effects on respiration as long as the H+
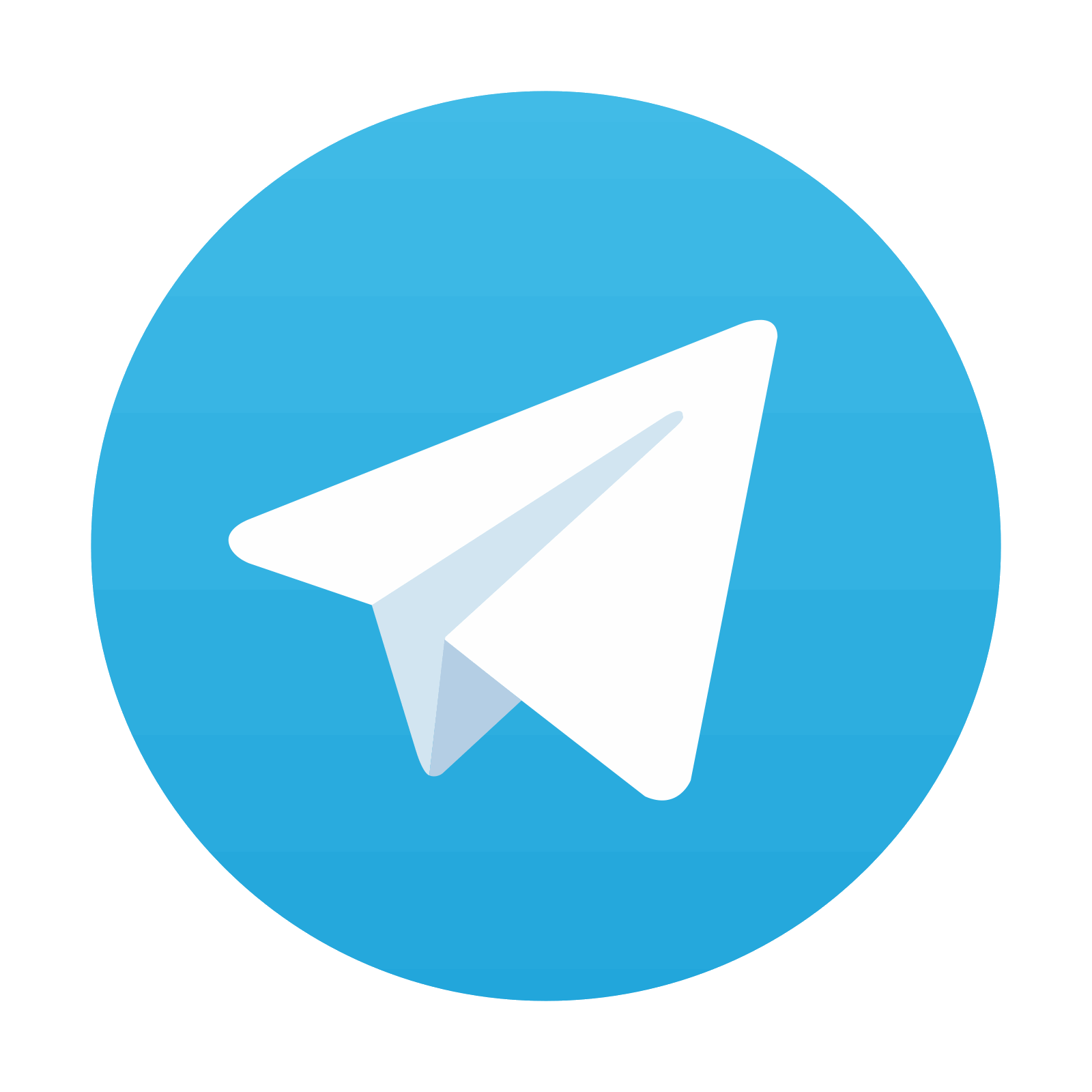
Stay updated, free articles. Join our Telegram channel
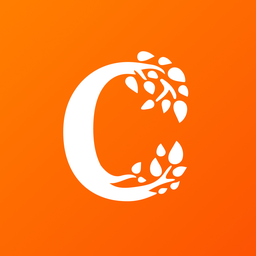
Full access? Get Clinical Tree
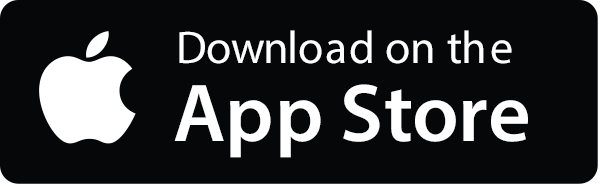
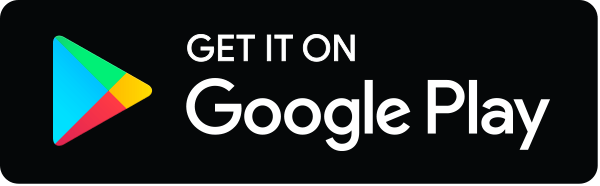
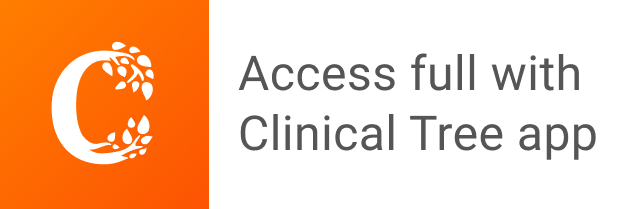