Potential Treatments for SARS-CoV-2 Beyond Current and Related New-Generation Antivirals and Monoclonal Antibodies
Roberto Patarca
William A. Haseltine
INTRODUCTION
Available vaccines, monoclonal antibodies, and antivirals for severe acute respiratory syndrome coronavirus 2 (SARS-CoV-2) infections have not kept pace with viral variation and have important limitations, ranging from mode and frequency of administration to loss of effectiveness and off-target effects. There is therefore an urgent need for novel strategies and tactics to deal with surveillance, detection, prevention, and treatment of SARS-CoV-2 infections.
Biomedical researchers have been reexploring approaches tested on other viruses or deciphering potential new ones. These approaches are broad ranged and directed at host cell or viral targets and influencing one or more stages of the viral life cycle. Virus-directed approaches offer the advantages of Paul Ehrlich’s “magic bullet,” affecting mainly the virus with minimal off-target effects on the host. In contrast, host-directed therapies target host pathways and processes hijacked by the virus for its replication, thereby indirectly restricting viral pathogenesis. Treatments targeting the cell itself may be more broadly effective against various viruses and variants thereof and alleviate the pressures leading to downstream drug resistance through viral mutations and selection.
Gene manipulation approaches targeting host and viral sequences are being explored and optimized to act in a tissue-specific and transient manner, thereby minimizing untoward off-target tissue effects. Other interventions involve repurposed or novel drugs targeting viral or host components, whereas some approaches refine small molecules for increased effectiveness. A variety of other therapeutic approaches are at a more incipient stage. However, most of the studies have been conducted in cell culture or animal models, and extrapolation to humans is at best hypothesis generating because of greater systemic complexity and interindividual variation in humans. Moreover, therapeutic approaches that were tested in other viruses decades ago have not made it to the clinic thus far.
This chapter summarizes therapeutic approaches aimed at breaking the viral life cycle at various stages from viral entry to exit and transmission. The availability of more approaches would foster the institution of more effective or multipronged interventions to help render viral resistance development less likely with fewer off-target effects and easier mode of administration. Formidable barriers, from infrastructure to cost, policy and inequity, must be overcome to implement the therapeutic approaches that are proven effective in humans.
NUCLEIC ACID–BASED THERAPEUTICS
Nucleic acid therapeutic technologies have become crucial platforms for rapid response to emerging viral threats.1 Vaccination using messenger (m)RNAs encoding viral antigens and monoclonal antibody therapies have joined a growing body of antiviral RNA interference (RNAi) and antisense oligonucleotide (ASO) approaches,2, 3, 4, 5, 6, 7, 8, 9, 10 and 11 as well as strategies involving Clustered Regularly Interspaced Short Palindromic Repeats (CRISPR)/Cas,12,13 which may be rapidly engineered to target viral genomes and/or transcripts once their sequences are known. However, various challenges remain for these technologies, including issues of specificity, biostability, immunogenicity, target site accessibility, delivery, and the potential for viral mutational escape. An example of how tissue specificity was overcome for the CRISPR-Cas approach is provided in the section on CRISPR-Cas13 targeting cathepsin L gene in lung tissue. As another example of overcoming limitations, in a study of small interfering RNAs (siRNAs), which are artificially synthesized 19- to 23-nucleotides-long double-stranded RNAs14 targeting SARS-CoV-2, siRNAs were not active against intermediate negative-sense transcripts. Targeting common sequences shared by all viral transcripts allowed simultaneous suppression of genomic and subgenomic RNAs by a single siRNA. The most effective suppression of viral replication and spread, however, was achieved by siRNAs that targeted open reading frame (ORF)1, which only exists in genomic RNA.2
Modular nucleic acid catalysts capable of sequence-specific RNA transesterification, including ribozymes, DNAzymes, and xeno (X)NAzymes, cleave target mRNAs with high specificity, thereby offering an alternative platform for the generation of an almost unlimited variety of precision antivirals. However, despite the development of a range of variants of the classic DNAzyme with the 10-23 catalytic core15 retargeted to viral RNAs,16, 17 and 18 including catalysts targeting the RNA genome of SARS-CoV-1,19 no antiviral DNAzyme has achieved clinical approval, and overall, nucleic acid
catalysts have shown limited efficacy in clinical applications. A limitation of DNAzymes is their dependence on concentrations of divalent metal ions outside the physiologic range: the 10-23 catalytic core requires concentrations of magnesium greater than 10 mM to fully fold into its active state,20 whereas at magnesium concentrations of 0.5 to 1 mM, DNAzyme activity is significantly reduced.21 The fact that when exogenously delivered into cells in vivo, DNAzymes, with or without an active catalytic core, have RNA knockdown effects22,23 might be secondary to their induction of RNase H and/or cytotoxic effects.22,24
catalysts have shown limited efficacy in clinical applications. A limitation of DNAzymes is their dependence on concentrations of divalent metal ions outside the physiologic range: the 10-23 catalytic core requires concentrations of magnesium greater than 10 mM to fully fold into its active state,20 whereas at magnesium concentrations of 0.5 to 1 mM, DNAzyme activity is significantly reduced.21 The fact that when exogenously delivered into cells in vivo, DNAzymes, with or without an active catalytic core, have RNA knockdown effects22,23 might be secondary to their induction of RNase H and/or cytotoxic effects.22,24
XNAzymes
DNAzymes can be modified with xeno-nucleic acids (XNAs), that is, synthetic alternative bases, sugars, and backbones,25, 26, 27 and 28 to generate XNAzymes that target SARS-CoV-2 and used as a viral biosensor29 or for the treatment of infection.30,31
The modular “FR6_1” XNAzyme, composed of 2′-deoxy-2′-fluoro-β-D-arabino nucleic acid, originally selected to cleave a sequence in the RNA genome of the Zaire Ebola virus, cleaves greater than 5-kb-long structured RNA under physiologic conditions in vitro and in vivo.32 Retargeted to cleave BRAF and KRAS mRNAs longer than 5 kb at physiologic magnesium concentrations with allelic selectivity for tumor-associated (BRAF V600E and KRAS G12D) mutations, the highly specific modular RNA endonuclease XNAzyme FR6_1 overcomes the activity limitations of previous DNA- and XNAzymes.32
Site-specific RNA endonuclease XNAzymes targeting SARS-CoV-2 ORF1ab, ORF7b, spike-, and nucleocapsid-encoding RNAs were further engineered to self-assemble into a catalytic nanostructure with enhanced biostability. This XNA nanostructure cleaves genomic SARS-CoV-2 RNA under physiologic conditions, and when transfected into cells inhibits SARS-CoV-2 infection by RNA knockdown. Therefore, XNAzymes could provide a platform for rapid generation of antiviral reagents.33 Off-target effects, however, remain to be evaluated.
Beyond the potential for reduced disruption of host regulatory networks,34 a combined XNAzyme strategy may avoid viral mechanisms for blocking silencing machinery35 and possibly enable to extend RNA knockdown to subcellular compartments or extracellular vesicles in which host effectors are scarce or unavailable, such as replication organelles or exosomes involved in cell-to-cell transfer of viral components.36 Targeting multiple RNA sequences using a cocktail of XNAzymes or a multifunctional construct renders emergence of escape variants less likely, thereby circumventing that seen when targeting single sites of rapidly mutating RNA viruses such as human immunodeficiency virus (HIV)-1.37
Noncoding RNAs (ncRNAs) also offer a wealth of therapeutic targets for a range of diseases; however, secondary structures and high similarity within sequence families render specific knockdown challenging. As another development of improved gene-silencing agents, a series of XNAzymes also composed of 2′-deoxy-2′-fluoro-β-D-arabino nucleic acid were synthesized to cleave individual ncRNA family members specifically or preferentially under quasi-physiologic conditions, including members of the classic microRNA cluster miR-17~92 (oncomiR-1) and the Y RNA hY5. Self-assembly of three anti-miR XNAzymes into a biostable catalytic XNA nanostructure targeted the cancer-associated microRNAs miR-17, miR-20a, and miR-21.38 These developments exemplify how XNAzymes can be further engineered for precision knockdown of specific RNAs, both coding and noncoding, with the potential to reduce off-target effects compared with other nucleic acid technologies.
CRISPR-Cas13d
Virologic and proteomic studies have revealed how SARS-CoV-2 hijacks the host cell machinery during infection,39,40 offering new guidance on the development of host-directed therapies. Genome-wide CRISPR-Cas9 screening for host factors identified cathepsin L (CTSL) as a top-ranked gene for infection by SARS-CoV-2, SARS-CoV-1, and Middle East respiratory syndrome (MERS)-CoV in cell models.41,42 In fact, most coronavirus spike proteins uncommonly undergo mutations within the CTSL cleavage sites (S1/S2 junction and S2′ position).43,44
Cathepsins play important physiologic roles in immune response, autophagy, and development, among other cellular processes.45,46 To avoid using CRISPR-Cas9 to permanently delete the CTSL gene, a chemically engineered lipid nanosystem encapsulating CRISPR-Cas13d was developed to
specifically and transiently knock down lung protease CTSL mRNA using a gene therapy strategy.47 The system blocked SARS-CoV-2 (including Delta variant) and SARS-CoV-1 infection in mice; decreased lung CTSL expression in normal mice efficiently, specifically, and safely; and extended the survival of mice lethally infected with SARS-CoV-2, correlating with decreased lung virus burden, expression of proinflammatory cytokines/chemokines, and severity of pulmonary interstitial inflammation. Postinfection treatment by this nanosystem dramatically lowered the lung virus burden and alleviated virus-induced pathologic changes.47 The selective knockdown of the CTSL mRNA expression by CRISPR-Cas13d in the lungs impairs the functions of both the catalytic and noncatalytic domains of CTSL. Inhibition of noncatalytic domains of proteases is important because they may enhance substrate specificity, steer cellular localization, and alter kinetic properties as well as the sensitivity to endogenous inhibitors.48
specifically and transiently knock down lung protease CTSL mRNA using a gene therapy strategy.47 The system blocked SARS-CoV-2 (including Delta variant) and SARS-CoV-1 infection in mice; decreased lung CTSL expression in normal mice efficiently, specifically, and safely; and extended the survival of mice lethally infected with SARS-CoV-2, correlating with decreased lung virus burden, expression of proinflammatory cytokines/chemokines, and severity of pulmonary interstitial inflammation. Postinfection treatment by this nanosystem dramatically lowered the lung virus burden and alleviated virus-induced pathologic changes.47 The selective knockdown of the CTSL mRNA expression by CRISPR-Cas13d in the lungs impairs the functions of both the catalytic and noncatalytic domains of CTSL. Inhibition of noncatalytic domains of proteases is important because they may enhance substrate specificity, steer cellular localization, and alter kinetic properties as well as the sensitivity to endogenous inhibitors.48
In contrast to the promising results with CRISPR-Cas13d approach for the inhibition of CTSL, several small-molecule CTSL inhibitors that had been reported to block entry in vitro of coronaviruses including SARS-CoV-2 and -140,43,49, 50 and 51 and pseudotyped SARS-CoV-2 infection in vivo44 only inhibit the active sites of the CTSL enzyme, are systematically distributed, and their antiviral activities are lost or significantly reduced by cell-surface expression of transmembrane trypsin-like serine protease 2 (TMPRSS2).40,52 Moreover, a potent CTSL inhibitor against SARS-CoV-1 infection in vitro failed to provide survival benefit in a lethal SARS-CoV-1 mouse model.51 It remains unclear whether the lack of antiviral potency of CTSL inhibitors in vivo and in some in vitro models is due to the use of alternative coronavirus entry pathways and/or to drug efficacy/delivery.
Ribonuclease Targeting Chimeras
Proteolysis targeting chimeras (PROTACs) bind to their target protein using a guide arm as “bait,” whereas the effector arm recruits an endogenous E3 ubiquitin ligase, resulting in polyubiquitination and subsequent proteasomal degradation of the target protein.54 This chimeric degrader concept has been extended to the RNA field in the form of ribonuclease targeting chimeras (RIBOTACs).6,55 RIBOTACs use synthetic small molecules that recognize secondary or tertiary RNA structures or ASOs that recognize RNA primary sequence as a “bait” fragment or guide arm, whereas the effector arm recruits the endogenous ribonuclease (RNase) L, causing degradation of the target RNA without affecting the host transcriptome.55, 56, 57 and 58
A study identified a series of compounds bound to the attenuator hairpin in the RNA element involved in programmed-1 ribosomal frameshift. These compounds were used as the guide arm for the RIBOTAC modality, and one named C5-RIBOTAC reduced SARS-CoV-2 RNA levels in a cellular model.59 RIBOTACs targeting the spike or envelope protein coding RNA using a 15-nucleotide complementary ASO as the guide arm and a 2′,5′-linked tetra-adenylate (2-5A4) as an RNase L recruiter reduced viral titer in infected Vero E6 cells.60 RNA-targeting molecules will probably synergistically inhibit viral replication when combined with protein-targeting drugs in cocktail therapies,6 which is also important to minimize development of viral resistance.
Locked Nucleic Acid–Containing Antisense Oligonucleotides
Oligonucleotides are nucleic acid polymers that can be used to treat or manage multiple diseases. Although the majority of oligonucleotide therapeutics have focused on gene silencing, other strategies are being pursued, including splice modulation and gene activation, to expand the range of possible targets beyond what is generally accessible to conventional pharmaceutical modalities. Most oligonucleotide modalities interact with their cognate target molecules via complementary Watson-Crick base pairing, rendering interrogation of the putative target sequence relatively straightforward. Highly specific lead compounds can often be rationally designed based on knowledge of the primary sequence of a target gene alone and lead candidates identified by rapid screening. By contrast, conventional small-molecule pharmaceuticals require much larger, and often iterative, screening efforts followed by extensive medicinal chemistry optimization. In addition, the use of oligonucleotides allows for precision and/or personalized medicine approaches as they can theoretically be designed to selectively target any gene with minimal, or at least predictable, off-target effects. Furthermore,
it is possible to target patient-specific sequences that cause rare diseases.61 Specific alleles, such as single-nucleotide polymorphisms or expanded repeat–containing mutant transcripts, can be preferentially targeted without silencing the wild-type mRNA62, 63, 64 and 65; distinct transcript isoforms66; pathogenic fusion transcripts (eg, Bcr-Abl67); traditionally “undruggable” targets (eg, proteins that may lack hydrophobic pockets that may accommodate a small molecule that also inhibits protein activity)68,69; and viral sequences that evolve resistance to an oligonucleotide therapy (ie, oligonucleotide design is modified to compensate for acquired escape mutations).70
it is possible to target patient-specific sequences that cause rare diseases.61 Specific alleles, such as single-nucleotide polymorphisms or expanded repeat–containing mutant transcripts, can be preferentially targeted without silencing the wild-type mRNA62, 63, 64 and 65; distinct transcript isoforms66; pathogenic fusion transcripts (eg, Bcr-Abl67); traditionally “undruggable” targets (eg, proteins that may lack hydrophobic pockets that may accommodate a small molecule that also inhibits protein activity)68,69; and viral sequences that evolve resistance to an oligonucleotide therapy (ie, oligonucleotide design is modified to compensate for acquired escape mutations).70
In addition to their ability to recognize specific target sequences via complementary base pairing, nucleic acids can interact with proteins through the formation of three-dimensional secondary structures—a property that is also being exploited therapeutically. For example, nucleic acid aptamers are structured nucleic acid ligands that can act as antagonists or agonists for specific proteins.71 Similarly, guide RNA molecules contain hairpin structures that bind to exogenously introduced Cas9 protein and direct it to specific genomic DNA loci for targeted gene editing.72
Locked nucleic acid–containing ASOs (LNA ASOs), which rely on Watson-Crick base pairing to target specific complementary RNA sequences, can be quickly designed to target any viral or host RNA sequence, including noncoding structural elements that may be important for viral replication. Depending on the design, LNA ASOs may induce target RNA degradation by recruiting RNase H for cleavage (gapmers) or act through steric hindrance (mixmers) via high-affinity binding to complementary targets.73 LNA ASOs are typically well tolerated, and a number of ASO therapeutics have received regulatory approval for clinical use in the treatment of cytomegalovirus retinitis, homozygous familial hypercholesterolemia, Duchenne muscular dystrophy, spinal muscular atrophy, hereditary transthyretin amyloidosis, and polyneuropathy.74 Additionally, ASO manufacturing is well established and can be readily scaled up, with chemically modified gapmer and mixmer ASOs containing interspersed LNA and DNA nucleotides linked by phosphorothioate bonds conferring increased affinity, stability, and improved pharmacokinetic/pharmacodynamic properties.75, 76 and 77
The stem-loop (SL)1 in the 5′-untranslated region (UTR) of SARS-CoV-2 allows the nonstructural protein (NSP)1 to switch infected cells from host to SARS-CoV-2 translation, and therefore presents a therapeutic target against coronavirus disease 2019 (COVID-19) that is conserved among immune-evasive variants. Targeting SL1 with LNA ASOs inhibits viral translation and makes the SARS-CoV-2 5′-UTR vulnerable to NSP1 suppression, hindering viral replication in vitro at a nanomolar concentration, as well as protecting against SARS-CoV-2-induced lethality in transgenic mice expressing human angiotensin-converting enzyme (hACE)2. This unique strategy of unleashing a virus’s own virulence mechanism against itself could force a critical trade-off between drug resistance and pathogenicity.78
A LNA ASO binding to the 5′ leader sequence of SARS-CoV-2, which is present in both genomic and subgenomic viral RNAs, disrupts a highly conserved SL structure with nanomolar efficacy in preventing viral replication in human cells.79 Daily intranasal administration of this LNA ASO in a COVID-19 mouse model suppressed viral replication in the lungs by over 80-fold and was efficacious for all SARS-CoV-2 “variants of concern” tested both in vitro and in vivo. Therefore, inhaled LNA ASOs targeting SARS-CoV-2 might reduce or prevent viral transmission and decrease COVID-19 severity. LNA ASOs are chemically stable and can be flexibly modified to target different viral RNA sequences and could be stockpiled for future epidemics of coronaviruses or other respiratory disease viruses.79
The highly conserved element involved in programmed-1 ribosomal shift, required for balanced expression of viral proteins, is a particularly attractive SARS-CoV-2 RNA target.6,80 The 6.9 Å resolution cryo-electron microscopy (EM) structure of this frameshift element (88 nucleotides, ~28 kDa), validated through an RNA nanostructure tagging method, presents a topologically complex fold in which the 5′-end is threaded through a ring formed inside a three-stem pseudoknot. ASOs developed guided by the latter structure impair frameshift stimulatory element (FSE) function in frameshifting assays and knock down SARS-CoV-2 virus replication in A549-ACE2 cells at 100 nM concentration.81
Another potential target of LNA ASOs is the stem-loop 2 motif (s2m), which is a regulatory mobile element with an unusually high degree of sequence conservation in the 3′-UTR in the genomes of many astroviruses, in which some picornaviruses and noroviruses, and coronaviruses, including SARS-CoV-2 and SARS-CoV-1, were originally identified.82, 83 and 84 The evolutionary conservation and
its occurrence in all viral subgenomic transcripts imply a key role for s2m in the viral infection cycle. The element, although stably folded, can nonetheless be invaded and remodeled spontaneously by ASOs that initiate pairing in exposed loops and trigger efficient sequence-specific RNA cleavage in reporter assays. ASOs also act to inhibit replication in an astrovirus replicon model system in a sequence-specific, dose-dependent manner and inhibit SARS-CoV-2 replication in cell culture.8 The s2m sequence is highly conserved in clinical isolates from patients that have tested positive for SARS-CoV-2 during the current pandemic; however, there are a few isolated exceptions.85 SARS-CoV-2 variants devoid of the s2m element seem to have been short lived, indicating that they were less evolutionarily fit than their s2m-containing counterparts. On a species level, however, there do not appear to be any losses and this pattern strongly suggests that the s2m element is essential to virus replication in SARS-CoV-2 and related viruses.86
its occurrence in all viral subgenomic transcripts imply a key role for s2m in the viral infection cycle. The element, although stably folded, can nonetheless be invaded and remodeled spontaneously by ASOs that initiate pairing in exposed loops and trigger efficient sequence-specific RNA cleavage in reporter assays. ASOs also act to inhibit replication in an astrovirus replicon model system in a sequence-specific, dose-dependent manner and inhibit SARS-CoV-2 replication in cell culture.8 The s2m sequence is highly conserved in clinical isolates from patients that have tested positive for SARS-CoV-2 during the current pandemic; however, there are a few isolated exceptions.85 SARS-CoV-2 variants devoid of the s2m element seem to have been short lived, indicating that they were less evolutionarily fit than their s2m-containing counterparts. On a species level, however, there do not appear to be any losses and this pattern strongly suggests that the s2m element is essential to virus replication in SARS-CoV-2 and related viruses.86
A potential enhancer element in SARS-CoV-2 might constitute another possible target for nucleic acid–based therapies. In coronaviruses, a good correlation has been observed between subgenomic RNA levels and the free energy of transcription regulatory sequence (TRS)-leader (L) and complementary TRS-B (body) duplex formation. The only documented exception is the subgenomic RNA encoding for the nucleocapsid (N) of the transmissible gastroenteritis coronavirus, the most abundant subgenomic RNA during viral infection in spite of its minimum free energy associated with duplex formation. This led to research87 that identified a novel transcription regulation mechanism operating in coronaviruses by which a 9-nucleotide sequence located 449 nucleotides upstream of the N gene TRS core sequence interacts with a complementary sequence just upstream of the TRS core sequence, specifically increasing the accumulation of subgenomic RNA encoding N. Alteration of this complementarity in mutant replicon genomes showed a correlation between the predicted stability of the base pairing between 9-nucleotide sequences and the accumulation of subgenomic RNA encoding N. This interaction is exclusively conserved in canine coronavirus and feline infectious peritonitis virus, the only coronavirus subgroup in which the N gene is followed by the group-specific gene 7 downstream at the 3′-end of the genome, whereas in the other coronavirus groups, including sarbecoviruses and arteriviruses, the N gene is the most distal gene in the genome. This is the first time that a long-distance RNA-RNA interaction regulating transcriptional activity specifically enhancing the transcription of one gene was described to occur in coronaviruses.87 However, the involvement in this process of other factors, such as higher-order RNA structure or protein factor(s), cannot be excluded. In fact, in the tombusvirus enhancer system, optimal subgenomic RNA transcription requires the coordinated activity of several distinct and highly integrated RNA elements that form a multicomponent RNA-based control system.88
Although the 9-nucleotide sequence (AUUACAUAU) and upstream complementary sequences (intermediate: AUACGUAAU and distal: AUAUGUAAU) identified in transmissible gastroenteritis virus (TGEV) upstream of N and within the M gene are not present in the genome of SARS-CoV-1 as reported by Moreno et al in 2008 or in that of MERS-CoV, both sequences (9-nucleotide: AUUACAUAU, nucleotides 21338 to 21346 in Wuhan reference strain; and more distal sequence: GGAUGUAAU, nucleotides 21280-21288) are present in SARS-CoV-2 only proximal to the S gene within the coding sequence for NSP16 at the end of ORF1b (Patarca and Haseltine, personal communication). One may posit that this potential RNA-RNA pairing with or without other RNA or protein interactions might differentially regulate expression of the S gene in SARS-CoV-2, contributing to its increased infectivity relative to that of SARS-CoV-1 or MERS-CoV, a hypothesis that beckons experimental testing.
Small Interfering RNAs
The RNA genome of SARS-CoV-1 is highly susceptible to RNAi,89, 90 and 91 particularly when delivered to the lungs of primates.92 siRNAs are short double-stranded RNA (dsRNA) molecules that induce gene silencing at the transcriptional or posttranscriptional level and can be delivered to the lungs via intranasal or intravenous routes.93,94 Upon entering the cytosol, siRNAs interact with several proteins to form an RNA-induced silencing complex (RISC) and subsequently knock down the expression of target genes based on sequence complementarity.
Lipid nanoparticles (LNPs) are used as delivery vehicles and are formulated to be stable in serum, circulate for long periods of time, and protect siRNA payloads from nucleases. These liposomes can be formulated based on alterations of size and composition to traffic to the lung.93,94 Prior to
the pandemic, the only U.S. Food and Drug Administration (FDA)-approved LNP-based therapy was the siRNA-LNP drug Patisiran (Onpattro) used for the treatment of polyneuropathy caused by hereditary transthyretin-mediated amyloidosis.95
the pandemic, the only U.S. Food and Drug Administration (FDA)-approved LNP-based therapy was the siRNA-LNP drug Patisiran (Onpattro) used for the treatment of polyneuropathy caused by hereditary transthyretin-mediated amyloidosis.95
For SARS-CoV-2, a study identified siV1, which efficiently targets NSP-1. SiV1 inhibited SARS-CoV-2 replication in Vero E6 and Huh-7 cells by more than 99% and 97%, respectively, without toxicity or induction of type I or III interferon (IFN) production, and its target sequence is highly conserved in SARS-CoV-2 variants.5 For HIV-1, single siRNA targeting leads to viral resistance emergence,96 whereas combinations of siRNAs hamper it.97
Intranasal instillation of unmodified naked siRNA inhibits respiratory viral infection in mice without the help of any carrier or transfection reagent98; therefore, intranasal and aerosol inhalation of naked siRNA have been widely utilized for delivering siRNA into pulmonary cells.99,100 ALN-RSV01 is an example of a successful application of an unmodified naked siRNA administered by nasal spray or aerosol inhalation to treat respiratory syncytial virus (RSV) infection. A phase 2 clinical trial showed that pretreatment with ALN-RSV01 significantly reduces the prevalence of RSV infection,101 and therapeutic treatment with ALN-RSV01 reduces the risk of bronchiolitis obliterans syndrome in lung transplant patients infected with RSV.102
Intranasal delivery of naked siRNA also has been proven effective to prevent and treat SARS-CoV-1 infection in nonhuman primates (Rhesus macaque).92 The inhaled and highly specific C6G25S siRNA targets a highly conserved RdRp region of SARS-CoV-1 and SARS-CoV-2 and includes 2′-O-methyl and 2′-F modifications, which reduce vulnerability to nuclease degradation and immune stimulation, including TLR-dependent and TLR-independent immune responses, that had been shown for naked siRNAs.103,104
C6G25S potently inhibited infection of various SARS-CoV-2 strains through an RNAi mechanism that specifically cleaves complementary viral RNA at the C6G25S recognition site.3 C6G25S inhibited dominant strains, including Alpha, Delta, Gamma, and Epsilon, at picomolar ranges of IC50 in vitro, as well as almost all strains at the time of the study. In K18-hACE2-transgenic mice, prophylactic treatment with C6G25S completely inhibited the production of infectious virions in lungs, and therapeutic treatment decreased 96.2% of virions resulting in significant prevention of virus-associated extensive pulmonary alveolar damage, vascular thrombi, and immune cell infiltrations.3 C6G25S was highly specific and well tolerated in transcriptome-wide off-target analysis and animal toxicology studies.
miR-2911, a natural microRNA that inhibits SARS-CoV-2,105 has a predicted target site overlapping that of C6G25S, but it only reduces viral load by 72% and is unable to inhibit the alpha variant in an in vitro assay.3 Moreover, the C-U transversion of Alpha variants located at the ninth nucleotide in C6G25S targeting site is tolerated by siRNA recognition106 and can still be inhibited by C6G25S with an IC50 of 0.46 nM. This finding suggests that C6G25S is a much more promising therapeutic agent than miR-2911.3
One of the major off-target genes of C6G25S, CXCL5, is a chemotactic factor secreted by lung epithelial cells and has a participatory role in COVID-19-associated pathogenesis by induction of neutrophil infiltration and acute lung injury.107,108 These findings suggest that C6G25S might have a unique dual effect whereby it can simultaneously inhibit SARS-CoV-2 infection and reduce the risk of severe illness. However, despite the picomolar range of IC50 in vitro, a relatively high administered dose of C6G25S is needed.
REPURPOSED DRUGS
Although antiviral development has focused on disrupting the enzymatic activities of viral proteins, targeting host factors that the virus requires to complete its life cycle is also an attractive option. Relative to their hosts, viruses have substantially less coding space in their genomes and therefore use the host to enable viral replication. The major advantages of inhibiting a virus indirectly through an essential host factor are 2-fold: It has the potential to act broadly because many viruses may use the same host protein, and it is more resistant to evasion by viral mutations compared with direct targeting of the virus, which can rapidly select for resistant viral mutants. Whereas not all host factors are easily targetable, some well-characterized enzymes, such as protein kinases, are of high interest for
host-directed antivirals. An essential step in the development of host-directed therapeutics, however, is defining those host factors that are essential for key aspects of the viral replication cycle.
host-directed antivirals. An essential step in the development of host-directed therapeutics, however, is defining those host factors that are essential for key aspects of the viral replication cycle.
The screening of approved drugs to identify therapeutics for drug repurposing is an effective approach that has been used for many viral diseases, including SARS-CoV-2.109, 110, 111, 112, 113, 114, 115, 116 and 117 Proteomic/chemoinformatic analyses help identify drug targets, novel agents, and potential drug repurposing that might perturb the viral-human interactome, while providing a mechanistic context to potential therapeutic interventions. Network systems biology allows to define the SARS-CoV-2-human protein-protein interactome, revealing interactions relevant to a range of functions. Examples include DNA replication (NSP1), epigenetic and gene expression regulators (NSP5, NSP8, NSP13, E), vesicle trafficking (NSP6, NSP7, NSP10, NSP13, NSP15, ORF3a, E, ORF8), lipid modification (spike), RNA processing and regulation (NSP8, N), ubiquitin ligases (ORF10), signaling (NSP8, NSP13, N, ORF9b), nuclear transport machinery (NSP9, NSP15, ORF6), cytoskeleton (NSP1, NSP13), mitochondria (NSP4, NSP8, ORF9c), and extracellular matrix (NSP9).39
Potential therapeutic interventions based on the aforementioned functions include inhibition of lysosomal acidification and trafficking with Bafilomycin A1, via inhibition of V-ATPase,118 and modulation of the endoplasmic reticulum stress and the protein unfolding response pathway by targeting the Sigma1 and Sigma2 receptor by drugs like haloperidol.39 Several human proteins in the interactome are targeted by drugs that have been proposed as candidate therapeutics for treating COVID-19, such as chloroquine,119,120 which targets the Sigma1 and Sigma2 receptors at mid and low millimolar concentrations, respectively. However, clinical studies of hydroxychloroquine, a known lysosomal acidification inhibitor and a less toxic derivative of chloroquine,121 and chloroquine indicate that they neither have antiviral efficacy nor improve clinical course or mortality.122, 123 and 124
Similarly, the antibiotic azithromycin, which has been proposed for COVID-19 treatment, has off-target activity against human mitochondrial ribosomes, components of which (MRPS5, MRPS27, MRPS2, and MRPS25) interact with the SARS-CoV-2 NSP8 protein. Nonetheless, a systematic review and meta-analysis of all types of clinical studies in 17 publications found no clinical improvement in patients with COVID-19.125 Other antibiotics that also have an off-target effect on mitochondrial ribosomes include chloramphenicol, tigecycline, and linezolid.39,126,127
Systematic genetic validation using genetic-based approaches128,129 will be key to determine the functional relevance of these interactions and if the human proteins are being used by the virus or are fighting off infection, because agents identified could be either detrimental or beneficial for infection. For instance, the HDAC2 inhibitors may compound the potential action of the NSP5 protease to hydrolyze this human protein.39
The Antineoplastic Obatoclax
Obatoclax was originally developed as a broadly acting B-cell lymphoma (BCL)-2 homology domain 3 inhibitor for cancer treatment130 by inducing apoptosis and promoting autophagy,130,131 each potentially virucidal outcomes. By combining pharmacologic evaluation and cellular network prioritization, obatoclax was identified as a potent inhibitor of SARS-CoV-2.132 Obatoclax consistently showed potent antiviral activity across in vitro systems, as well as efficacy in the mouse disease model. The 10-fold decrease in lung titers is promising because a similar reduction of virus load has been associated with decreased mortality in patients with COVID-19.133 The latter activity of obatoclax does not appear to be driven via BCL-2 inhibition because seven other BCL-2 inhibitors that were tested were weak or inactive. In turn, obatoclax, but not other BCL-2 antagonists, appeared to inhibit endosomal acidification, which also likely inhibited alphavirus infection by preventing pH-dependent endosomal escape.134 SARS-CoV-2 requires endosomal acidification to trigger fusion of endosomal and virus membranes to release the virus capsid and genome into the cell cytosol and initiate replication. Consistent with this mechanism, the pseudotype infection assay showed that obatoclax partially blocks infection for the SARS-CoV-2 pseudotype by affecting endosomal activity.135 However, another report showed that the influenza virus, which also requires endosomal acidification to infect cells, was unaffected by obatoclax.136
The data discussed support a dominant role for obatoclax interfering with S function and therefore endosomal escape, but also suggest an additional entry-independent mechanism. In fact, obatoclax executes a double-strike against SARS-CoV-2. It prevents SARS-CoV-2 entry by blocking endocytosis of virions through diminished endosomal acidification and the corresponding inhibition
of the enzymatic activity of the endosomal cysteine protease cathepsin L. Additionally, obatoclax impairs SARS-CoV-2 S-mediated membrane fusion by targeting the myeloid cell leukemia (MCL)-1 protein and reducing furin protease activity. In accordance with these overarching mechanisms, obatoclax blocked the virus entry mediated by S proteins derived from several SARS-CoV-2 variants of concern including Alpha (B.1.1.7), Beta (B.1.351), and Delta (B.1.617.2). Obatoclax as a novel effective antiviral compound may thus keep SARS-CoV-2 at bay by blocking both endocytosis and membrane fusion.135
of the enzymatic activity of the endosomal cysteine protease cathepsin L. Additionally, obatoclax impairs SARS-CoV-2 S-mediated membrane fusion by targeting the myeloid cell leukemia (MCL)-1 protein and reducing furin protease activity. In accordance with these overarching mechanisms, obatoclax blocked the virus entry mediated by S proteins derived from several SARS-CoV-2 variants of concern including Alpha (B.1.1.7), Beta (B.1.351), and Delta (B.1.617.2). Obatoclax as a novel effective antiviral compound may thus keep SARS-CoV-2 at bay by blocking both endocytosis and membrane fusion.135
The Antihelminthic and Molluscicide Niclosamide
Using two high-content microscopy-based screenings with more than 3,000 approved drugs to search for inhibitors of spike-driven syncytia, a study137 identified 83 drugs that inhibited S-mediated cell fusion, with several belonging to defined pharmacologic classes. Among drugs that also protected against virus replication and associated cytopathicity, one of the most effective was the antihelminthic138 and molluscicide139 drug niclosamide, which markedly blunts calcium oscillations and membrane conductance in spike-expressing cells by suppressing the activity of TMEM16F (also known as anoctamin 6). TMEM16F is a calcium-activated ion channel and scramblase that is responsible for exposure of phosphatidylserine on the cell surface140 and whose levels are further increased upon exposure to S.
The activation of members of the TMEM16 family by SARS-CoV-2 S might have specific relevance for COVID-19 pathogenesis,137 as it might participate in inflammation (TMEM16A promotes nuclear factor [NF]-κB activation and interleukin [IL]-6 secretion),141 thrombosis (TMEM16F is essential for lipid scrambling in platelets during blood coagulation),142,143 dysfunction of endothelial cells,144 and alveolar edema and diarrhea through increased chloride secretion. Niclosamide is active against SARS-CoV-2 and various enveloped and nonenveloped viruses.112 Although this drug has relatively low solubility, there is evidence of considerable absorption, with serum levels reaching 1 to 20 μM.145,146
Besides niclosamide, other drugs known to inhibit the anoctamin/TMEM16 family also scored positive in inhibiting S-induced syncytia in assays, including nitazoxanide, hexachlorophene, and dichlorophen.137 Gefitinib, which is known to block TMEM16A-activated epidermal growth factor receptor (EGFR) on the plasma membrane,147 also inhibited S-driven fusion. In addition, it appears likely that, like niclosamide and nitazoxanide,148 other drugs that target TMEM16A, in particular trifluoperazine,149 serotonin reuptake inhibitors,150,151 and ivermectin,152 all of which scored positive against syncytia, might also inhibit TMEM16F. However, ivermectin failed in multiple COVID-19 clinical trials.153, 154, 155, 156, 157, 158, 159 and 160
The Antihypertensive Carvedilol and the Antineoplastic Toremifene
A study161 leveraged high-throughput yeast two-hybrid and tandem mass tag affinity purification followed by mass spectrometry to generate a binary and co-complex SARS-CoV-2-human protein-protein interactome network. The study then prioritized host-targeting therapies by searching FDA-approved and investigational drugs for their potential anti-SARS-CoV-2 effect using state-of-the-art network proximity methods. Analyzing two large independent databases of patients with COVID-19, the study found that use of the antihypertensive carvedilol was associated with a lowered risk (17%-20%) of a positive COVID-19 test. Experimental validation showed that carvedilol inhibits SARS-CoV-2 infection with a half-maximal effective concentration of 4.1 μM. Carvedilol, as well as the antihypertensive hydrochlorothiazide, may directly inhibit viral entry by disrupting the spike-ACE2 protein-protein interaction.161
In the aforementioned analysis,161 the antineoplastic drug toremifene, a selective estrogen receptor modulator, achieved significantly closer network proximity among the top 23 candidates determined and has a desired anti-SARS-CoV-2 profile. Toremifene efficiently blocks infection by SARS-CoV-2,112 SARS-CoV-1,110 MERS-CoV,162 and Ebola virus by inhibiting virus entry after internalization, suggesting that it does not work through classical pathways associated with the estrogen receptor.113 Toremifene may inhibit SARS-CoV-2 cell entry by blocking the S and NSP14 proteins,163 consistent with functional assay data on spike-ACE2 protein-protein interaction, SARS-CoV-1 or MERS-CoV pseudotyped particle entry, and 3CL enzymatic activity.161
The Antidepressant Fluoxetine
The selective serotonin reuptake inhibitor (SSRI) fluoxetine blocks SARS-CoV-2 replication efficiently.164, 165, 166 and 167 In clinical studies its use was associated with lower risk of death or intubation in patients
hospitalized for COVID-19.168 Fluoxetine was also associated with a 26% reduction in relative risk of mortality among patients infected with SARS-CoV-2.169 Fluoxetine affects the activity of sphingomyelinase, which is optimal at a pH of 4.5 to 5.0. SSRIs are protonated within the lysosome, which blocks their rediffusion into the cytoplasm. Their local accumulation causes detachment, and thereby inactivation of acid sphingomyelinase from the membrane of lysosomal intraluminal vesicles.170 This also applies to acid ceramidase, which catalyzes the breakdown of lysosomal ceramides, released upon acid sphingomyelinase activity, into sphingosine and fatty acids, thereby balancing ceramide levels in lysosomes and also ensuring the subsequent export of sphingosine from this compartment after conversion into sphingosine-1-phosphate.170 However, a novel functionalized fluoxetine derivative did not prevent SARS-CoV-2 infection, but impaired acid ceramidase activity, followed by the entrapment of viral particles in lysosomes, which points to acid ceramidase as a potential SARS-CoV-2 host factor.171
hospitalized for COVID-19.168 Fluoxetine was also associated with a 26% reduction in relative risk of mortality among patients infected with SARS-CoV-2.169 Fluoxetine affects the activity of sphingomyelinase, which is optimal at a pH of 4.5 to 5.0. SSRIs are protonated within the lysosome, which blocks their rediffusion into the cytoplasm. Their local accumulation causes detachment, and thereby inactivation of acid sphingomyelinase from the membrane of lysosomal intraluminal vesicles.170 This also applies to acid ceramidase, which catalyzes the breakdown of lysosomal ceramides, released upon acid sphingomyelinase activity, into sphingosine and fatty acids, thereby balancing ceramide levels in lysosomes and also ensuring the subsequent export of sphingosine from this compartment after conversion into sphingosine-1-phosphate.170 However, a novel functionalized fluoxetine derivative did not prevent SARS-CoV-2 infection, but impaired acid ceramidase activity, followed by the entrapment of viral particles in lysosomes, which points to acid ceramidase as a potential SARS-CoV-2 host factor.171
Ursodeoxycholic Acid and Z-Guggulsterone
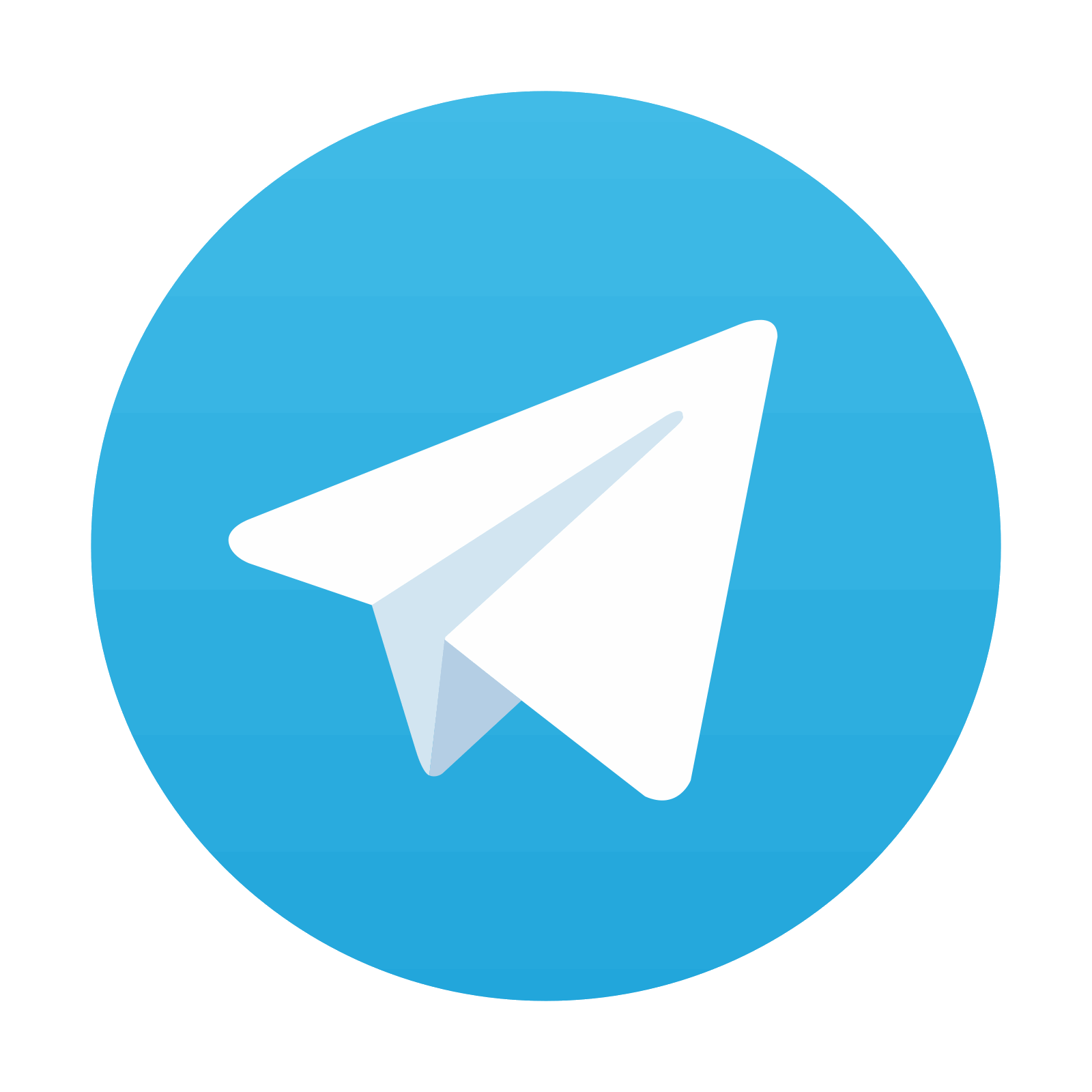
Stay updated, free articles. Join our Telegram channel
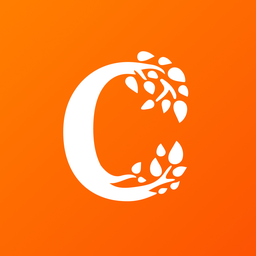
Full access? Get Clinical Tree
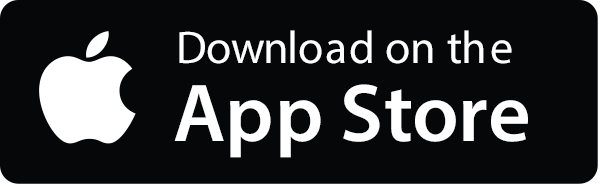
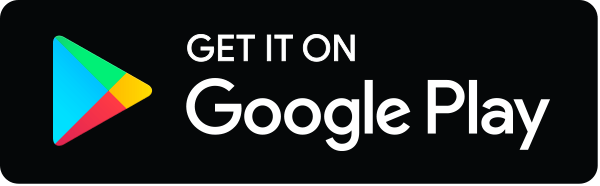
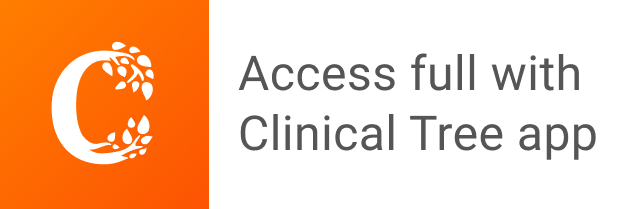