KEY POINTS
Plastic surgery is the field of surgery that addresses congenital and acquired defects, striving to return form and function.
Plastic surgery has been a field of innovation. The future of the specialty likely includes advancements in the areas of regenerative medicine, fetal surgery, and reconstructive transplantation with composite tissue allotransplants.
Children diagnosed with cleft and craniofacial anomalies benefit from interdisciplinary care at a specialized center focusing on team care. Long-term follow-up during growth and development is critical for optimal outcomes.
Reconstructive surgery attempts to restore form and function through techniques that include skin grafting, use of muscle flaps, bone grafting, tissue expansion, free tissue transfer with microsurgery, and replantation.
Aesthetic surgery is surgery performed to reshape the normal structure of the body to improve the patient’s appearance and self-esteem. Patients undergoing aesthetic surgery present a unique challenge. The most important outcome parameter is patient satisfaction, and therefore, a thorough understanding of the patient’s motivations, goals, and expectations is critical.
HISTORICAL BACKGROUND
The field of plastic surgery focuses on the restoration of form and function to those who have congenital and acquired deformities. Plastic surgery routinely addresses novel problems and challenges; therefore, the plastic surgeon must have an expert knowledge of anatomy and surgical technique to address new challenges.
The word plastic is derived from the Greek plastikos, meaning “to mold.” Although the term plastic surgery can be found in several medical writings from the eighteenth and nineteenth centuries, it was John Staige Davis who established the name of the specialty with the 1919 publication Plastic Surgery—Its Principles and Practice.
One of the earliest accounts of reconstructive surgery can be found in the Sushruta Samhita, an early text from the sixth or seventh century b.c. by the practitioner Sushruta. In this writing, the reconstruction of an amputated nose with a pedicled forehead flap and the reconstruction of the ear with cheek flaps were described. In addition, in the first century a.d., the Roman physicians Aulus Cornelius Celsus and Paulus Aegineta described operations for facial reconstruction.
The first textbook of plastic surgery is believed to be Gaspara Tagliacozzi’s 1597 publication De Curtorum Chirurgia per Insitionem. This text describes the reconstruction of the nose with a pedicled arm flap. The nineteenth century saw advances in reconstructive surgery, including Giuseppe Baronio’s successful grafting of sheepskin. The techniques for perfecting human skin grafting followed later in the nineteenth century.
Great advances in plastic surgery occurred as a result of the first and second world wars. Out of the fields of dental surgery, otolaryngology, ophthalmology, and general surgery, the discipline of plastic surgery was established. The founders of the field include Sir Harold Gillies, an otolaryngologist who established a center for the treatment of maxillofacial injuries in England; V. H. Kazanjian, a dental surgeon from Boston, who established a center in France for the treatment of facial injuries incurred in World War II; and Vilray P. Blair, from St. Louis, who established centers for the treatment of soft tissue and maxillofacial reconstruction for the U.S. Army. With the onset of World War II, centers of excellence for hand reconstruction appeared as well.
In the last 50 years, advances in plastic surgery have included the transplantation of both autologous and allogeneic tissue, tissue expansion, regional muscle and myocutaneous flap transfers, distant transfer of free flaps using microsurgery, replantation of traumatically amputated extremities and digits, and the emergence of craniofacial surgery. The future of plastic surgery will likely see further advances in the realms of regenerative medicine, fetal surgery, and reconstructive transplantation.
GENERAL PRINCIPLES
Human skin exists in a state of tension created by internal and external factors. Externally, skin and underlying subcutaneous tissue are acted on by gravity and clothing. Internally, skin is subjected to forces generated by underlying muscles, joint extension and flexion, and tethering of fibrous tissues from zones of adherence. As a result, when skin is incised linearly, it gapes to variable degrees. When a circular skin excision is performed, the skin defect assumes an elliptical configuration paralleling the lines of greatest tension. Carl Langer, an anatomist from Vienna, first fully described these tension lines in the mid-1800s based on his studies of fresh cadavers.1 A. F. Borges described another set of skin lines that, different from Langer’s lines, reflect the vectors of relaxed skin tension.2 Although the term Langer’s lines often is used interchangeably with the term relaxed skin tension lines, the former lines describe skin tension vectors observed in the stretched integument of cadavers exhibiting rigor mortis, whereas the latter lines lay perpendicular to and more accurately reflect the action of underlying muscle2 (Fig. 45-1). Relaxed skin tension lines may be exploited to create incisions that minimize anatomic distortion and improve cosmesis. In areas of anatomic mobility, such as the neck or over joints, incisions are oriented less for aesthetic reasons and more with the goal of avoiding scar contractures and subsequent functional compromise. In general, incisions are placed perpendicular to the action of the joint.
There are situations, however, in which the direction of the incision has been preestablished, as in acute lacerations, burns, or old contracted and distorting scars. In these circumstances, the principles of proper incision placement can be combined with simple surgical techniques to reorient the scar and lessen the deformity. The Z-plasty technique uses the transposition of random skin flaps both to break up a linear scar and to release a scar contracture through lengthening (Fig. 45-2; Table 45-1). W-plasty is the technique of scar excision and reconstruction in zigzag fashion to camouflage the resulting scar.
The fundamentals of plastic surgery are based on wound healing physiology. Wound repair consists of an exquisitely regulated symphony of molecular and cellular instruments that act in concert to restore the local tissue environment to prewound conditions. Metabolic imbalances in the wound milieu drive this orchestration and continue to direct it until healing resolves the disturbance. Although a detailed review of wound physiology is presented elsewhere in this text, it is useful to emphasize several points.
Tissue injury disrupts the tissue microenvironment and sets into motion a cascade of events that combine to reestablish the environmental status quo. Disrupted blood vessels fill the wound space with red blood cells and plasma. Injured cells release factor III (thromboplastin), which accelerates the clotting cascade. Clotting factors in the plasma are activated, and the coagulation cascade forms thrombin and eventually fibrin. Simultaneously, the complement system activates and produces chemoattractive complement protein fragments. Platelets, activated by thrombin and exposed collagen, release a number of growth factors and cytokines. Traumatized vessels contract in response to both direct physical stimulation and prostaglandins released by platelets. Intact local microvasculature vasodilates and leaks plasma in response to inflammatory mediators such as histamine, kinins, and serotonin. These early events, and others, establish inflammation and, finally, homeostasis.3
Platelet activation initiates the first major escalation in the inflammatory response. Within minutes, platelets release signaling molecules from their α-granules to attract macrophages, polymorphonuclear cells (PMNs), fibroblasts, and vascular endothelial cells. Within a few hours of injury, PMNs and macrophages invade the wound and remove tissue debris, coagulation proteins, and bacteria. Although both PMNs and macrophages begin to marginate early, PMNs dominate during the first few days. PMNs also constitute the primary defense against invading organisms that have breached the epithelial barrier. PMNs and macrophages, in concert with the complement system, form the basis of “natural” or “nonspecific” immunity. If there is no infection or foreign material, the neutrophil population diminishes by the second day, whereas macrophages continue to amass.3
Macrophages become the major population by the third day after injury. These cells then dominate the wound region for days to weeks. Macrophages are the “masterminds” behind the finely tuned array of repair events that characterizes the proliferative phase of healing. Like neutrophils, activated macrophages continue the task of wound débridement. They are a rich source of degradative enzymes that process the extracellular matrix to make room for remodeling. Tightly coordinated release of the many growth factors, colony-stimulating factors, interleukins, interferons, and cytokines gives the macrophage the ability to regulate migration, proliferation, and specific protein synthesis of multiple cell lines. Macrophages lead the procession of new tissue into the wound dead space. Immature, replicating fibroblasts follow the macrophages. Mature fibroblasts then advance into the wound and are, in turn, followed by newly forming capillary buds, the last cells in the procession.3
Thus, injury perturbs the microenvironment and leads to the autoamplifying inflammatory phase. As a result of these processes, three changes occur in the wound: the environment becomes hypoxic, acidotic, and hyperlactated. One biochemical pathway by which this low redox potential state can signal cells to take biologic action is the adenosine diphosphoribose (ADPR) system. Recent evidence has shown that alterations of the polyADPR system affect regulation of collagen and vascular endothelial growth factor (VEGF) transcription.3 Consequently, the metabolic state that is so deranged in the wound microenvironment is intimately linked to altered cellular function and leads to reparative cell phenotypes.
After inflammation has begun, fibroblasts are attracted by many stimuli and then proliferate and migrate into the site of injury. Fibroblasts are the major producers of collagen in the repair response. Substances that increase collagen deposition and maturation include lactate, oxygen, and growth factors. Lack of these agents as well as steroid treatments decrease collagen in wounds.
Macrophages also usher along angiogenesis, largely through the release of VEGF. VEGF production is upregulated by the same wound metabolic environment that stimulates collagen production. As neovascularization takes place, many of the conditions that signaled the start of the inflammatory and proliferative phases are resolved, and the wound healing response recedes.
Epidermal cells are attracted to the healing wound by the same cytokines that attract other wound cells. Epithelialization proceeds best in a moist environment with high oxygen tension.3
Preoperative, intraoperative, and postoperative interventions may be taken by the surgeon to minimize infection and optimize wound healing (Tables 45-2–45-4). These measures all draw on what we understand of the physiologic wound healing process.
• Assess and optimize cardiopulmonary function; correct hypertension. |
• Treat vasoconstriction: attend to blood volume, thermoregulatory vasoconstriction, pain, and anxiety. |
• Assess recent nutrition and provide treatment as appropriate. |
• Treat existing infection. |
• Assess wound risk using the SENIC index. |
• Start administration of vitamin A in patients taking glucocorticoids. |
• Maintain tight blood glucose control. |
• Administer appropriate prophylactic antibiotics at start of procedure. Keep antibiotic levels high during long operations. |
• Keep patient warm. |
• Maintain gentle surgical technique with minimal use of ties and cautery. |
• Keep wounds moist. |
• Perform irrigation in cases of contamination. |
• Elevate tissue oxygen tension by increasing the level of inspired oxygen. |
• Delay closure of heavily contaminated wounds. |
• Use appropriate sutures (and skin tapes). |
• Use appropriate dressings. |
• Keep patient warm. |
• Provide analgesia to keep patient comfortable, if not pain free. |
• Keep up with third-space losses. Remember that fever increases fluid losses. |
• Assess perfusion and react to abnormalities. |
• Avoid diuresis until pain is gone and patient is warm. |
• Assess losses (including thermal losses) if wound is open. |
• Assess need for parenteral/enteral nutrition and respond. |
• Continue to control hypertension and hyperglycemia. |
Skin is comprised of 5% epidermis and 95% dermis. The dermis contains sebaceous glands, whereas sweat glands and hair follicles are located in the subcutaneous tissue. The dermal thickness and concentration of skin appendages vary widely from one location to another on the body. The skin vasculature is superficial to the superficial fascial system and parallels the skin surface. The cutaneous vessels branch at right angles to penetrate subcutaneous tissue and arborize in the dermis, finally forming capillary tufts between dermal papillae.4
Skin grafting techniques date back >3000 years to India, where forms of the technique were used to resurface nasal defects in thieves who were punished for their crimes with nose amputation. Modern skin grafting methods include split-thickness grafts, full-thickness grafts, and composite tissue grafts (Table 45-5). Each technique has advantages and disadvantages. Selection of a particular technique depends on the requirements of the defect to be reconstructed, the quality of the recipient bed, and the availability of donor site tissue.
TYPE | DESCRIPTION | THICKNESS (IN) |
---|---|---|
Split thickness | Thin (Thiersch-Ollier) | 0.006–0.012 |
Intermediate (Blair-Brown) | 0.012–0.018 | |
Thick (Padgett) | 0.018–0.024 | |
Full thickness | Entire dermis (Wolfe-Krause) | Variable |
Composite tissue | Full-thickness skin with additional tissue (subcutaneous fat, cartilage, muscle) | Variable |
Split-thickness skin grafting represents the simplest method of superficial reconstruction in plastic surgery. Many of the characteristics of a split-thickness graft are determined by the amount of dermis present. Less dermis translates into less primary contraction (the degree to which a graft shrinks in surface area after harvesting and before grafting), more secondary contraction (the degree to which a graft shrinks during healing), and better chance of graft survival. Thin split grafts have low primary contraction, high secondary contraction, and high reliability of graft take, often even in imperfect recipient beds. Thin grafts, however, tend to heal with abnormal pigmentation and poor durability compared with thick split grafts and full-thickness grafts. Thick split grafts have more primary contraction, less secondary contraction, and may take less hardily. Split grafts may be meshed to expand the surface area that can be covered. This technique is particularly useful when a large area must be resurfaced, as in major burns. Meshed grafts usually also have enhanced reliability of engraftment, because the fenestrations allow for egress of wound fluid and excellent contour matching of the wound bed by the graft. The fenestrations in meshed grafts reepithelialize by secondary intention from the surrounding graft skin. The major drawbacks of meshed grafts are poor cosmetic appearance and high secondary contraction. Meshing ratios used usually range from 1:1.5 to 1:6, with higher ratios associated with magnified drawbacks.
By definition, full-thickness skin grafts include the epidermis and the complete layer of dermis. The subcutaneous tissue is carefully removed from the deep surface of the dermis to maximize the potential for engraftment. Full-thickness grafts are associated with the least secondary contraction upon healing, the best cosmetic appearance, and the highest durability. As a result, they are frequently used in reconstructing superficial wounds of the face and the hands. These grafts require pristine, well-vascularized recipient beds without bacterial colonization, previous irradiation, or atrophic wound tissue.
Skin graft take occurs in three phases: imbibition, inosculation, and revascularization. Plasmatic imbibition refers to the first 24 to 48 hours after skin grafting, during which time a thin film of fibrin and plasma separates the graft from the underlying wound bed. It remains controversial whether this film provides nutrients and oxygen to the graft or merely a moist environment to maintain the ischemic cells temporarily until a vascular supply is reestablished. After 48 hours, a fine vascular network begins to form within the fibrin layer. These new capillary buds interface with the deep surface of the dermis and allow for transfer of some nutrients and oxygen. This phase, called inosculation, transitions into revascularization, the process by which new blood vessels either directly invade the graft or anastomose to open dermal vascular channels and restore the pink hue of skin. These phases are generally complete by 4 to 5 days after graft placement. During these initial few days, the graft is most susceptible to interference in engraftment caused by infection, mechanical shear forces, and hematoma or seroma.4
Composite tissue grafts are donor tissue containing more than just epidermis and dermis. They commonly include subcutaneous fat, cartilage and perichondrium, and muscle. Although less common than skin grafts, grafts of this type are particularly useful in select cases of nasal reconstruction. Excision of the thick skin of the nasal lobule may create too deep a defect to reconstruct with a full-thickness skin graft. The ear lobe composite graft provides thicker coverage with good color match and a fairly inconspicuous donor site (Fig. 45-3). Similarly, the root of the helix of the ear may be used to reconstruct the alar rim, providing skin coverage, cartilaginous support, and internal lining in a single technique.
Figure 45-3.
Composite graft reconstruction of nasal lobule. A. Scarred lobule from previous lesion excision. B. Scar excision markings. C. Insetting of composite ear lobe skin and subcutaneous fat graft. D. Postoperative day 3; note the pink hue of revascularization. E. Appearance at 5 weeks postoperatively. F. Donor site at 5 weeks postoperatively.
A flap is a vascularized block of tissue that is mobilized from its donor site and transferred to another location, adjacent or remote, for reconstructive purposes. The difference between a graft and a flap is that a graft brings no vascular pedicle and derives its blood flow from recipient site revascularization, whereas a flap arrives with its blood supply intact.
Random pattern flaps have a blood supply based on tiny blood vessels in the dermal-subdermal plexus, as opposed to the discrete, well-described vessels of axial pattern flaps (Fig. 45-4).5 Random flaps are typically used to reconstruct relatively small, full-thickness defects that are not amenable to skin grafting. Unlike axial pattern flaps, random flaps are limited by their geometry. The generally accepted reliable length-to-width ratio for a random flap is 3:1. Exceptions to this rule abound, however. There are many different types of random cutaneous flaps that differ in geometry and mobility. A transposition flap is rotated about a pivot point into an adjacent defect (Fig. 45-5). A Z-plasty is a type of transposition flap in which two flaps are rotated, each into the donor site of the other, to achieve central limb lengthening (see Fig. 45-2). Another common transposition flap is the rhomboid (Limberg) flap (Fig. 45-6). Rotational flaps are similar to transposition flaps but differ in that they are semicircular (Fig. 45-7). Advancement flaps slide forward or backward along the flap’s long axis. Two common variants include the rectangular advancement flap and the V-Y advancement flap (Fig. 45-8). Like transposition flaps, interpolation flaps rotate about a pivot point. Unlike transposition flaps, they are inset into defects near, but not adjacent, to the donor site. An example of an interpolation flap is the thenar flap for fingertip reconstruction (Fig. 45-9).
Figure 45-4.
Random pattern flap architecture. a. = artery. (Reproduced with permission from Aston et al.5)
Figure 45-8.
Random pattern advancement flap. A. Rectangular advancement flap with Burow’s triangle excision. B. V-Y advancement flap. (Reproduced with permission from Aston et al.5)
Figure 45-9.
Random pattern interpolation flap—the thenar flap. A. Middle fingertip injury with exposed bone and tendon. B. Elevation of distally based random pattern thenar flap. C. Insetting. D and E. Function and form at 3 months, after skin grafting of donor site. (Photographs reproduced with permission from M. Gimbel.)
The composition of a flap describes its tissue components. For example, a cutaneous flap contains skin accompanied by a variable amount of subcutaneous fat. A fasciocutaneous flap contains skin and fascia, whereas an adipofascial flap contains subcutaneous fat and fascia without overlying skin. A muscle flap contains muscle only, whereas a myocutaneous flap also contains the overlying skin and intervening tissues. An osseous flap contains vascularized bone only, whereas an osteomyocutaneous flap contains, in addition, muscle, skin, and subcutaneous tissues.
The contiguity of a flap describes its position related to its source. Local flaps are transferred from a position adjacent to the defect. Regional flaps are from the same anatomic region of the body as the defect (e.g., the lower extremity region or the head and neck region). Distant flaps are transferred from a different anatomic region to the defect. They may remain attached to the source anatomic region (pedicled flaps) or may be transferred as free flaps by microsurgery. These are completely detached from the body, and their blood supply is reinstated by microvascular anastomoses to recipient vessels close to the defect.
The term pedicle was originally used to describe a bridge of tissue that remained between a flap and its source, similar to how a peninsula remains attached to its mainland. However, as knowledge of flap blood supply and (micro)vascular anatomy has improved over the years, the term pedicle has increasingly become reserved for describing the blood vessels that nourish the flap. Thus its current use is, in essence, vascular pedicle in shortened form. As a refinement, it is possible to dissect the pedicle free of its surrounding tissues (termed skeletonization) to allow any tortuosity of the supplying blood vessels to be released in order to maximize their reach toward a given defect. This is usually performed in a retrograde direction starting from where the pedicle enters the flap tissues. Similarly, it is possible to detach the desired skin paddle circumferentially from all unneeded surrounding tissues in order to maximize the freedom with which the flap can be inset to reconstruct the defect. Hence, a pedicled island flap has had its cutaneous component circumferentially incised while preserving its vascular pedicle. Therefore, a free flap and a pedicled island flap differ only in that the former requires transection of the vascular pedicle for anastomosis to alternative recipient vessels, whereas the pedicle of the latter remains in continuity.
Such flaps that are supplied by an anatomically defined configuration of vessels are described as having an axial pattern blood supply and can be transferred as local, regional, or distant, and pedicled, island pedicled, or free flaps.6 Arising from the aorta are arteries that supply the internal viscera and other deep vessels that divide to form the main arterial supplies to the trunk, head, and extremities. They ultimately feed interconnecting vessels that supply the vascular plexuses of the fascia, subcutaneous tissue, and skin. These interconnecting vessels reach the skin via either fasciocutaneous (also called septocutaneous) vessels that traverse fascial septae between muscles, musculocutaneous perforators that penetrate muscle bellies, or direct cutaneous vessels that traverse neither muscle bellies nor fascial septae.7 Axial pattern flaps, incorporating suprafascial tissues, are supplied by these fasciocutaneous (septocutaneous), musculocutaneous, or direct cutaneous arteries. The internal viscera are also a source of axial pattern flaps, such as the jejunum flap and omentum flap. The circulation of bone- and muscle-containing flaps also is mainly axial in pattern. It also is possible to design local flaps, such as V-Y advancements and rhomboid flaps, as axial pattern flaps. In contrast to axial pattern flaps, random pattern flaps are only commonly transferred as local flaps by virtue of their lack of a defined vascular pedicle and cannot be transferred as island pedicled or free flaps. Axial pattern flaps may possess some areas with random pattern circulation, usually located at the flap periphery.
The volume of tissue reliably vascularized by the pedicle of an axial pattern flap defines its limits. In other words, the portion of a flap that extends beyond the capabilities of its vascular pedicle to perfuse it reliably will ordinarily undergo necrosis of that portion. This can be clarified conceptually. The arterial tree can be described in terms of its angiosomes.8 An angiosome is a block of tissue that is reliably supplied by a given artery. Neighboring angiosomes overlap, just as the dermatomes of neighboring nerves overlap. An anatomic angiosome is defined by the limits of an artery’s ramifications, where it forms anastomoses with a neighboring anatomic angiosome. The vessels that pass between these anatomic angiosomes are called choke vessels. In life, these may open or close in response to physiologic changes to increase or decrease, respectively, an artery’s dynamic angiosome momentarily. Accordingly, at any given time point, the dynamic angiosome of an artery may be approximated by the volume of tissue stained by an intravascular administration of fluorescein into that artery (indicating the reach of blood flow from that artery into tissues). The potential angiosome of an artery is the volume of tissue that can be included in a flap that has undergone conditioning (see below). Both the dynamic and potential angiosomes extend beyond the anatomic angiosome of an artery. Although the angiosomal concept provides some guidance to the size and volume limits of a flap harvest, there remains no quantifiable method to predict safe flap harvest limits exactly.
Conditioning refers to any procedure that increases the reliability of a flap by enlarging the angiosome of the pedicle artery from its dynamic toward its potential angiosome. Invoking the delay phenomenon, for example, has improved the survival of flaps that otherwise would more frequently be complicated by unpredictable partial necrosis, such as the pedicled transverse rectus abdominis myocutaneous (TRAM) flap. The procedure can be particularly useful in patients at higher risk, such as those who are obese, smoke, or have received radiotherapy. One method of delay for the pedicled TRAM flap is to divide a major portion of its blood supply, the deep inferior epigastric artery on both sides, approximately 2 weeks before transfer. In response, blood from the anatomic angiosome of the superior epigastric artery appears to flow into that of the interrupted deep inferior epigastric artery via intervening choke vessels. As a result, the flap becomes conditioned to rely on the superior epigastric artery. The TRAM flap can then be transferred based on the superior epigastric artery with less risk of its distal portions becoming ischemic and possibly necrotic. Several theories have been proposed to explain the delay phenomenon, including metabolic compensatory responses to relative ischemia and dilatation of choke vessels; however, its mechanisms remain incompletely understood.9
Further subclassifications of flap circulation have been introduced for muscular and fasciocutaneous flaps.10 Individual muscles have been classified by Mathes and Nahai into five types (I–V) according to their blood supply (Table 45-6). This classification is also applied to the respective myocutaneous flaps. Fasciocutaneous flaps also have been classified by these authors into types A, B, and C (Table 45-7). The inclusion of muscle in a flap may serve to increase flap bulk (so as to obliterate dead space) or to provide a functioning component with the harvest of its motor nerve for coaptation to a recipient motor nerve. The purported advantages of muscle-containing flaps over fasciocutaneous flaps for use in previously infected tissue beds or for fracture healing have been debated.
CLASSIFICATION | VASCULAR SUPPLY | EXAMPLE |
---|---|---|
Type I | One vascular pedicle | Gastrocnemius |
Type II | Dominant and minor pedicles (the flap cannot survive based only on the minor pedicles) | Gracilis |
Type III | Two dominant pedicles | Rectus abdominis |
Type IV | Segmental pedicles | Sartorius |
Type V | One dominant pedicle with secondary segmental pedicles (the flap can survive based only on the secondary pedicles) | Pectoralis major |
CLASSIFICATION | VASCULAR SUPPLY | EXAMPLE |
---|---|---|
Type A | Direct cutaneous vessel that penetrates the fascia | Temporoparietal fascial flap |
Type B | Septocutaneous vessel that penetrates the fascia | Radial artery forearm flap |
Type C | Musculocutaneous vessel that penetrates the fascia | Transverse rectus abdominis myocutaneous flap |
With progressive advancements in flap transfer techniques and in understanding of microvascular flap anatomy, plastic surgeons have steadily increased the number and variety of available flaps, thereby improving the results of flap reconstructions. In addition, this knowledge has reduced the morbidity associated with flap harvest. Perhaps the most important advancement in flap surgery within recent decades has been the introduction of the perforator flap.11 Perforator flaps evolved from the observation that the muscle component of myocutaneous flaps served as a carrier of blood vessels to the overlying fasciocutaneous tissues. Prior to this, it had been deemed mandatory to include the muscle for reliable harvest of fasciocutaneous tissues supplied by musculocutaneous perforators, even if it was not necessary to include that muscle for the reconstruction. This unfortunately caused an unnecessary muscular deficit at the donor site, and for this reason, fasciocutaneous flaps that were supplied by musculocutaneous perforators instead of septocutaneous vessels were sometimes abandoned. The introduction of intramuscular retrograde dissection techniques, however, allowed the skeletonization of a musculocutaneous perforator from its encasement within a muscle belly, which spared that muscle from flap harvest and preserved its donor site function.7,11 Further refinement of this concept gave rise to the harvest of cutaneous flaps based on any vessel that penetrated the fascia, which preserved the muscle (when the vessel was a musculocutaneous perforator) as well as the fascia (by suprafascial dissection). Within the last decade, free-style flap harvest has also been introduced.12 With a handheld Doppler ultrasound probe, the surgeon is able to identify an arterial supply to almost any area of skin with the desired reconstructive characteristics and trace that pedicle in retrograde fashion along whatever direction it takes, preserving donor site fascia and muscle as necessary. Although the exact definition of what a perforator flap is remains contentious, its advantages remain clear: reduced donor site morbidity, reduced flap bulk, and increased flexibility in choosing desired flap components for reconstruction. The circulation of perforator flaps is axial in pattern; consequently, they can be transferred as pedicled island flaps or by microvascular free tissue transfer.
A free tissue transfer, often referred to as a free flap procedure, is an autogenous transplantation of vascularized tissues. Any axial pattern flap with pedicle vessels of a suitable diameter can be transferred as a free flap. This involves three main steps: (a) complete detachment of the flap, with devascularization, from the donor site; (b) revascularization of the flap with anastomoses to blood vessels in the recipient site; and (c) an intervening period of flap ischemia. Flap circulation must be restored within a tolerable ischemia time.
Given the small diameter of most flap pedicle vessels (usually between 0.8 and 4.0 mm), these anastomoses are usually performed using an operative microscope that provides dedicated illumination and between 6× and 40× magnification. Any surgery performed with the aid of an operative microscope is termed microsurgery; such anastomoses are therefore termed microvascular anastomoses. High-magnification surgical loupes are usually used for flap harvest, especially for dissecting the flap pedicle, because they allow greater operator freedom. Aside from microvascular anastomosis, microsurgical techniques include microneural coaptation, microlymphatic anastomosis, and microtubular anastomosis.
The first successful free tissue transfer in humans was of a jejunal free flap for cervical esophagus reconstruction in 1957; however, the surgeons did not use microsurgery for the anastomoses. The first microvascular free tissue transfers in humans were carried out during the late 1960s and early 1970s. Free flaps were initially considered to be a last-resort option to reconstruct the most complex defects. However, as a result of improved microsurgical techniques and microinstrumentation, as well as proper patient and free flap selection and effective postoperative monitoring methods, the success rates have increased to exceed 95%.13 Today, free tissue transfer is often the first-choice treatment for many defects and is no longer considered the last-ditch effort. It is now ubiquitously used in appropriate patients by reconstructive plastic surgeons worldwide.
The predetermining factor in free flap failure is occlusion of its blood supply due to thrombosis. As enumerated by Virchow’s triad, any factors that alter normal laminar blood flow, cause endothelial damage, or change the constitution of blood (producing hypercoagulability) increase the risk of thrombosis (Table 45-8).14 Avoidance of this complication, therefore, begins with a thorough patient evaluation for the presence of acquired or inherited thrombophilic tendencies. The patient’s hemodynamic status influences that of the free flap and should be optimized. The effect of tobacco smoking on free flap success has been debated, with some larger retrospective studies reporting no difference in thromboembolic complications; however, smoking is well known to affect wound healing.13,15 Smoking and the use of potentially vasoconstrictive agents, such as caffeine, should be avoided for several weeks before and after a free flap procedure. The restoration of normal laminar blood flow and avoidance of endothelial damage are addressed principally by careful flap insetting and meticulous microvascular surgical technique.
ALTERED LAMINAR BLOOD FLOW | ENDOTHELIAL DAMAGE | HYPERCOAGULABILITY |
---|---|---|
Tension or intimal malalignment at the anastomosis site; twisting, kinking, compression, or vasospasm of pedicle vessels | Iatrogenic damage (e.g., back-walled anastomotic suture, poor vessel handling, too many sutures) | Acquired thrombophilic tendency (e.g., pregnancy, paraneoplastic Trousseau’s syndrome, antiphospholipid antibody syndromes) |
Intraluminal structures (e.g., atherosclerotic plaque, venous valves, back-walled anastomotic suture) | Previous vessel damage (e.g., atherosclerosis, trauma) | Hereditary thrombophilias (e.g., activated protein C resistance, protein C/protein S deficiency, hyperhomocysteinemia) |
Planning a free flap goes beyond a simple calculation of matching flap and defect dimensions and tissue characteristics. The surgeon must, in addition, consider several important technicalities: what flap pedicle length and size are required (affected by flap choice); which recipient vessels to use; how to orient anastomoses (end to end or end to side), deal with mismatched donor and recipient vessel dimensions, overcome unhealthy donor and/or recipient vessels (e.g., traumatic dissection, scarred surgical field due to previous operation or radiotherapy), inset flap tissues (to maximize functional and cosmetic results without detriment to flap circulation), route the pedicle (to restore normal blood flow without pedicle kinking, twisting, or compression), position the patient (especially if the flap is to be inset over mobile soft tissue or joints), and place postoperative dressings (so as to produce no compression of the flap or pedicle); and what donor site morbidity will likely result (there is a risk-benefit decision between defect severity and flap choice).16 In addition, the surgeon must have a suitable backup plan to overcome intraoperative troubles; for example, insufficient pedicle length can be addressed with an interpositional vein graft adjoining the donor and recipient vessels, and iatrogenic vessel injury or severely aberrant anatomy may necessitate use of a backup flap or backup recipient vessels.13
A clear understanding of the blood supply to the free flap and its tissue components is a prerequisite to harvesting a viable free flap. Pedicle vessels must be identified and protected and handled minimally and atraumatically to avoid thrombogenic factors (see Table 45-8). Meticulous technique also reduces the risk of vasospasm, but the latter can be ameliorated by topical lidocaine or papaverine should it occur. Critical vessels connecting flap components must also be recognized and preserved. Under microscope magnification, the donor and recipient vessels should be dissected back to health. The presence of, for example, venous valves, atherosclerotic plaques, intimal trauma, and intraluminal prolapse of adventitial tissue at or adjacent to the anastomosis site increases the risk of thrombosis. The vessel ends should be cleared of periadventitial tissues for 3 to 5 mm with sharp dissection under the microscope. Periadventitial dissection should be limited to this extent, so as to avoid potential devascularization of the vessel wall by removal of the vasa vasorum and prevent the subsequent delayed development of a perianastomotic pseudoaneurysm. Adventitiectomy also helps relieve vasospasm by increasing compliance of the vessel wall and by inducing a local sympathectomy effect. The vessel ends usually are stabilized with a double approximating microvascular clamp for anastomosis. Interrupted sutures or, less commonly, continuous sutures can accomplish the anastomosis. The microneedle typically has a three-eighths circle curvature and is between 30 and 150 μm in size. Its monofilament microsuture is usually between 9-0 and 11-0 caliber. The dimensions of the vessels to be anastomosed define the choice of microneedle and microsuture. Less commonly, suture alternatives such as fibrin adhesives or laser welding (these remain largely experimental) and mechanical anastomotic devices (e.g., venous couplers) may be used. Triangulating or bisecting suturing techniques can help to achieve an even placement of sutures. Normally, each suture should include the full thickness of both vessel walls, none should catch the opposite vessel wall (which causes disastrous luminal occlusion and intimal trauma), and the size of each bite should approximate the vessel wall thickness. The configuration of the anastomosis can be either end-to-end (Fig. 45-10), if the distal circulation can be adequately preserved, or end-to-side (Fig. 45-11), if the distal circulation must be preserved, as in the case of an extremity supplied by one dominant vessel. An end-to-side orientation may also be useful to overcome dramatically mismatched donor-recipient vessel dimensions. Whatever the method chosen, microanatomic differences between the vessels should be respected so as to achieve accurately approximated intimal surfaces in a tension-free anastomosis, devoid of redundancy that might promote kinking.13
The clinical monitoring of a free flap should start during flap harvest, especially before its pedicle is divided. A free flap that is struggling to maintain normal perfusion characteristics during harvest most likely has insufficient circulation, which may be due to arterial or venous compromise or a combination of both (Table 45-9). Flap compromise may be due to reversible factors such as pedicle kinking, tensioning, or twisting; patient hemodynamic compromise; or an overly large flap harvest for the chosen pedicle vessels. If poor flap perfusion continues despite the absence or correction of all these factors, an inherent flap problem or a critical vascular injury to the flap or its pedicle must be considered, and it may not be safe to continue its harvest. This is one example of a situation in which a backup plan may require execution.
CLINICAL SIGN | ARTERIAL COMPROMISE | VENOUS COMPROMISE |
---|---|---|
Color | Becoming paler | Increasingly reddish or purplish |
Temperature | Becoming cooler | Becoming warmer |
Tissue turgor | Reducing | Increasing |
Capillary refill time | Becoming slower | Becoming faster |
Pinprick bleeding | Increasingly sluggish | Quickening (and darkening) |
Clinical flap monitoring continues after successful restoration of arterial inflow and venous outflow. The mainstay of postoperative free flap monitoring is clinical assessment (see Table 45-9), although supplementary instrument monitoring also can be helpful. Doppler ultrasound assessment of arterial and venous signals is useful for monitoring buried or concealed flaps. If flap perfusion was healthy before division of its donor site pedicle, then poor perfusion after anastomoses is likely due to either a technical error or insufficient systemic hemodynamics. The latter usually is correctable by ensuring that the patient and the patient’s environment are suitably warm and by initiating intravenous colloid challenge or, if indicated, blood transfusion. Numerous potential technical errors, which have been described in the earlier paragraphs on planning and anastomosis technique, may occur. Routine postoperative patient monitoring includes measurement of total fluid inputs, urinary catheter output (which should be >1 mL/kg per hour), core temperature, and arterial blood pressure (systolic pressure should be >100 mmHg), as well as pulse oximetry. The patient and free flap are best monitored in an intensive care setting by experienced staff until both are stable enough for routine ward assessments.17
Occlusion of the anastomosis most commonly arises from intraluminal thrombosis or from external compression of the pedicle, such as from surrounding tissues, fluid accumulation (e.g., hematoma and tissue edema), or overly tight dressings or skin sutures. Because there is a threshold of ischemia beyond which a flap will sustain irreversible tissue and/or microcirculatory damage, it is important that the early signs of flap circulatory compromise be recognized as quickly as possible and the underlying problem diagnosed and corrected promptly if flap health is to be restored successfully.17 Different tissues tolerate differing durations of ischemia in correlation with their tissue-specific basal metabolic rate. Although cooling free flaps (to reduce basal metabolic rate) has a variably protective effect in experimental settings, it appears that this practice contributes little to improving free flap success in the clinical setting as long as warm ischemia times are kept to <4 hours for most tissues; exceptions include bowel flaps, which are more susceptible to ischemia.13
Given that the predisposing factor for free flap failure is thrombus formation, it is understandable that plastic surgeons have looked to anticoagulant therapies in an effort to improve success rates. Although such drugs, including the dextrans, aspirin, heparins, and also some fibrinolytics, appear beneficial in experimental settings, large clinical trials have failed to show any conclusive associations between their use and either free flap success or failure rates.18 It seems intuitive to use these drugs for failing free flaps as an adjunctive measure alongside operative reexploration and surgical intervention. The surgeon must be aware of their contraindications and recognize that their side effects, apart from bleeding, are occasionally serious. Venous congestion may be addressed by surgical measures as well as by application of medicinal Hirudo medicinalis leeches (with concomitant Aeromonas hydrophila prophylaxis) or by chemical “leeching” (topical heparin combined with dermal punctures).
Unfortunately, the “no-reflow” phenomenon is occasionally witnessed and leads to irreversible flap failure. This describes a situation in which no venous return drains into the pedicle vein of the flap, even though adequate arterial inflow passes the arterial anastomoses and is seen to enter the flap tissues. The no-reflow phenomenon sometimes follows an extended ischemic insult and appears to be a self-perpetuating cycle of endothelial cellular swelling, inflammatory vasoconstriction, impaired microcirculatory flow, stasis, microcirculatory thromboses, progressive ischemia, and flap failure.13
Despite these potential problems, free flap success rates exceed 95% in experienced hands. There is no doubt that increasing microsurgical experience is critical to improving free flap success rates. The laboratory setting is an excellent environment in which to progress beyond the early portion of one’s learning curve through supervised microsurgical training and execution of microvascular anastomoses and microvascular free flap procedures in small animals.
Although skin grafts and local flaps are very useful in reconstructing many superficial defects, they are not without their drawbacks. Both leave donor site defects with cosmetic and/or functional sequelae. Grafts are limited in color match and durability, whereas local flaps may supply insufficient tissue and produce contour irregularities. The advent of tissue expansion has created the potential to increase the amount of local, well-matched tissue that can be advanced or transposed as a flap while decreasing donor site morbidity.
The most common method of skin expansion involves the placement of an inflatable silicon elastomer balloon with an integrated or remote port beneath the skin and subcutaneous tissue followed by serial inflation with saline. After completion of expansion, usually over weeks to months, the expander is removed and the redundant overlying skin may be advanced into an adjacent defect. Expanders are available in a multitude of shapes and sizes that can be tailored to the reconstruction. In breast reconstruction, the tissue expander is replaced with a permanent implant instead of using the tissue as a flap to re-create the volume of the breast mound. Histologically, expanded skin demonstrates thickened dermis with enhanced vasculature and diminished subcutaneous fat. Studies have shown that the skin expansion is due not merely to stretch or creep but also to actual generation of new tissue.19
The technique of tissue expansion comes with its share of potential complications, including infection, hematoma, seroma, expander extrusion, implant failure, skin necrosis, pain, and neurapraxia. Furthermore, an inflated expander is a very visible, albeit temporary, deformity that may cause patients much distress.
Despite these imperfections, tissue expansion has become a major treatment modality in the management of giant congenital nevi, secondary reconstruction of extensive burn scars, scalp reconstruction, and breast reconstruction. The technique has permitted the plastic surgeon to perform reconstructions with tissue of similar color, texture, and thickness with minimal donor site morbidity.
PEDIATRIC PLASTIC SURGERY
Orofacial clefting is the most common congenital anomaly and is known to occur in 1 in 500 live white births.20 The incidence is lower in African Americans and higher in Native Americans and Asians. Clefting of the lip and/or palate is felt to occur around the eighth week of embryogenesis, either by failure of fusion of the medial nasal process and the maxillary prominence or by failure of mesodermal migration and penetration between the epithelial bilayer of the face. The cause of orofacial clefting is felt to be multifactorial. Factors that likely increase the incidence of clefting include increased parental age, drug use and infections during pregnancy, smoking during pregnancy, and a family history of orofacial clefting. The increased chance of clefting when there is an affected parent is approximately 4%.
The primary palate is defined as all tissue anterior to the incisive foramen, including the anterior hard palate (premaxilla), alveolus, lip, and nose. The secondary palate includes everything posterior to the incisive foramen, including the majority of the hard palate and the soft palate (velum). Clefting can involve the lip and nose, with or without a palatal cleft. Clefts of the lip and/or palate are first classified as unilateral or bilateral and then as complete or incomplete (Fig. 45-12). Complete clefts of the lip affect the entire lip and extend up into the nose. Incomplete clefts affect only a portion of the lip and contain a bridge of tissue connecting the central and lateral lip elements, referred to as Simonart’s band.
Considerable controversy remains over the details of the timing, technique, and protocol for treating children with orofacial clefting. The treatment protocol described in this chapter is accepted at many large cleft centers around the United States. All infants born with cleft-craniofacial anomalies benefit from care by a specialized team dedicated to the treatment of congenital anomalies. Today, this is widely accepted as the standard of care. Often, patients are seen prenatally after a diagnosis is made using sophisticated antenatal ultrasonography. The prenatal consultation has proven to be beneficial to parents, serving to dispel fears and uncertainties, and assuring them that treatment exists. After the infant’s birth, a team evaluation occurs, and input is obtained from the surgeon, speech and language pathologist, social worker, craniofacial orthodontist, geneticist, otorhinolaryngologist, and pediatrician. For infants born with orofacial clefting, initial concerns relate to successful feeding and breathing. Infants with palatal clefts cannot generate negative pressure when suckling and therefore need milk dispensed into their mouths from a specialized nurser when they make suckling motions.
Once adequate nutrition and a safe airway are ensured, attention is turned to the cleft anomaly. Attempts to lessen the deformity and set the stage for the surgical repair of the lip and nose begin with a process known as presurgical infant orthopedics (PSIO), which includes procedures such as nasoalveolar molding (NAM) (Fig. 45-13). NAM repositions the neonatal alveolar segments, brings the lip elements into close approximation, stretches the deficient nasal components, and turns wide complete clefts into the morphology of narrow “incomplete” clefts. After PSIO with NAM, the definitive single-stage cleft lip and nose repair is performed at 3 to 6 months of age. With this initial operation, the lip deformity is repaired and a primary nasoplasty reconstructs the cleft lip nasal deformity. If the family does not have access to PSIO or have the resources for this time-intensive therapy, a cleft lip adhesion can be performed as an initial stage in the repair. The preliminary cleft lip adhesion unites the upper lip and nasal sill, truly converting complete clefts into incomplete clefts. A cleft lip adhesion is performed in the first or second month of life, and the definitive cleft lip and nose repair follows at 4 to 6 months. After the definitive cleft lip and nose repair, the cleft palate is repaired in a single stage at 9 to 12 months of age.
The unilateral cleft lip is classically associated with a cleft lip nasal deformity. The cleft lip nasal deformity includes lateral, inferior, and posterior displacement of the alar cartilage. This results from the deficient and clefted underlying skeleton as well as the unopposed pull of the clefted orbicularis oris muscle abnormally inserted on the alar base (Fig. 45-14A). The maxillary minor segment (the smaller alveolar/maxillary segment on the clefted side) is collapsed medially. The process of unilateral cleft lip repair can be thought of as “philtral subunit reconstruction.” The goal of the operation is to level Cupid’s bow and reconstruct the central philtrum of the lip, ideally placing the incision and subsequent scar as close to the normal philtral column as possible. The surgical repair is performed under general anesthesia, and local anesthesia containing epinephrine is used. Many different techniques of cleft lip and nose repair have been proposed; however, most of the commonly used procedures are variations of a “rotation-advancement” procedure.21 The rotation-advancement procedure, as championed by Millard (Fig. 45-14B), rotates the philtral subunit of the central lip downward to level Cupid’s bow as the lateral lip element is advanced into the defect created by the downward rotation of the philtrum. Some surgeons choose to perform primary closure of the alveolar cleft at the time of primary lip and nose repair, called a gingivoperiosteoplasty. If the alveolar cleft is to be repaired, the gingivoperiosteoplasty is performed by raising mucoperiosteal flaps within the alveolar cleft margin and reapproximating them across the alveolar cleft defect. This creates a bony tunnel closed with periosteal flaps and facilitates the generation of bone in the alveolar defect. It is accepted today that some form of primary nasoplasty should be performed at the time of primary definitive lip repair. Techniques to release and reposition the nasal tip cartilages, as well as the ala, are performed with variations of tip rhinoplasties using suture methods. Some surgeons choose to use postoperative internal and/or external splints to maintain the nasal correction achieved at surgery during the healing process.
In the complete bilateral cleft lip and nose deformity, the central lip element, called the prolabium, is entirely separate from the rest of the upper lip. The prolabium is displaced on top of the central alveolar segment, called the premaxilla, containing the unerupted four central incisors. Often, the premaxilla and prolabium are outwardly displaced. This is referred to as a flyaway premaxilla. For the child with a complete bilateral cleft lip and nose, PSIO is a very important step in preparing the child for definitive lip and nose surgery by retracting the premaxilla into the maxillary arch, repositioning the lip segments, and stretching the rudimentary columella. Bilateral cleft lip and nose repairs often are versions of straight-line repairs, with the Mulliken technique being the more commonly performed (Fig. 45-15). In the bilateral cleft lip deformity, the new philtrum is made from the prolabium and is united to the lateral lip elements on top of the repaired orbicularis oris muscle.22
During the eighth to twelfth weeks of gestation, the mandible becomes more prognathic, the tongue drops from beneath the clefted lateral palatine processes, and the palatal shelves migrate upward into a more horizontal position and fusion occurs. A cleft palate results from the failure of fusion of the two palatal processes. As with labial clefting, isolated clefts of the palate are multifactorial in etiology, and isolated clefts of the palate are more likely to be associated with other anomalies. Between 8% and 10% of isolated clefts of the palate are associated with the 22q deletion of velocardiofacial syndrome.23
The main goal of cleft palate surgery is to help the patient attain normal speech, which results from velopharyngeal competence. During speech, the soft palate, or velum, is moved posteriorly and superiorly, primarily by the levator palatini muscle sling that suspends the velum from the skull base. Velopharyngeal competence is obtained during attempted speech when the velum approximates the posterior pharyngeal wall, preventing air and liquid from regurgitating into the nasal cavity. Velopharyngeal competence allows intraoral pressure to be built up for speech sounds. A cleft palate precludes this from occurring and results in velopharyngeal incompetence (VPI). Because it is impossible for the oral and nasal cavities to be partitioned in the patient with a cleft palate, it is also difficult for the patient to develop negative intraoral pressure for an effective suck. Therefore, specialized nursers are used to dispense liquid into the infant’s mouth during the suckling motions. Children with clefts of the palate have an increased incidence of otitis media; this may be related to the abnormality of the velar musculature and ineffective function of the eustachian tube. The increased incidence of otitis media can result in hearing loss if not treated appropriately. In addition, VPI and nasal air escape during speech results in hypernasal speech.
As with the repair of cleft lip and nose, the timing, technique, and protocols for cleft palate repair are controversial. Most agree that palate repair should be performed before the development of speech. The cleft palate usually is repaired when the infant is between 6 and 18 months of age. Cleft palate repair also is performed under general anesthesia, with the head slightly hyperextended and a retractor, such as the Dingman mouth gag, placed intraorally to retract the tongue and endotracheal tube. An epinephrine solution is injected into the palate. Techniques of hard palate closure include the use of unipedicled hard palate mucoperiosteal flaps as in the Wardill-Veau-Kilner repair or bipedicled hard palate mucoperiosteal flaps as in the von Langenbeck repair. Both the unipedicled and bipedicled hard palate palatoplasty techniques rely on the greater palatine neurovascular pedicle. Soft palate or velar closure techniques are divided into straight-line and Z-plasty procedures. With either a straight-line or Z-plasty velar repair, the levator palatini muscle should be independently repaired; this is called an intravelar veloplasty. The clefted levator is identified coursing sagittally in an anterior-posterior direction, abnormally inserted onto the posterior edge of the hard palate. In intravelar veloplasty, it is released from the posterior edge of the hard palate in the midline and dissected free from abnormal attachments to the aponeurosis of the tensor veli palatini muscle and superior constrictor laterally. After its complete release, the levator palatini muscle is united in the midline, with reconstruction of the levator muscle sling that suspends the velum from the skull base and aids in velopharyngeal competence.
The authors prefer the double opposing Z-plasty technique of soft palate or velar reconstruction known as the Furlow palatoplasty.24 The procedure uses four triangular flaps, two oral and two nasal, with the posteriorly based flaps containing the released levator muscles. The Z-plasty lengthens the soft palate, prevents longitudinal scarring from a straight-line repair, and produces a secondary pharyngoplasty effect by narrowing the velopharyngeal port (Fig. 45-16).
Complications of palatoplasty include wound healing problems resulting in a breakdown of the suture line and the development of a fistula. The literature reports fistula rates ranging from approximately 1% to 20%. Treatment of palatal fistulae is particularly challenging, because the recurrence rates have been noted to approach 96%. The second most common complication of palatoplasty is the incomplete correction of speech and the development of postoperative VPI. The literature reports postoperative VPI rates ranging from 10% to 40%. Some of the best rates of velopharyngeal competence have been reported with the Furlow double opposing Z-plasty palatoplasty. Postoperative VPI is treated with pharyngoplasty—either a posterior pharyngeal flap pharyngoplasty or a sphincter pharyngoplasty. A posterior pharyngeal flap is a static flap formed from the posterior pharyngeal wall including mucosa and a portion of the superior constrictor muscle. The midline superiorly based pharyngeal flap is inset into the posterior free edge of the soft palate, permanently attaching it to the posterior pharyngeal wall. The sphincter pharyngoplasty has been reported to involve creation of a dynamic sphincter made with the bilateral posterior tonsillar pillars containing the palatopharyngeus muscle. The superiorly based tonsillar pillars are elevated from the lateral pharynx and inset into a horizontal incision on the posterior pharyngeal wall at the level of the adenoid pad.
Craniofacial surgery is the subspecialty of plastic surgery dealing with hard and soft tissue deformities of the craniofacial skeleton, treating the congenital, developmental, and acquired defects of the cranial and/or facial skeleton. Craniofacial surgery addresses the functional and equally important appearance-related issues surrounding these deformities. Attempting to separate the functional impairment from the appearance-related issues is arbitrary, because it can be argued that the most important function of a face is to look like a face.25 Numerous studies have established the importance of facial form and the significant emotional impact that facial deformities have on a person’s life and sense of self.
The field of craniofacial surgery finds its origins in the aftermath of the world wars and the need to treat massive facial injuries. In 1967, Dr. Paul Tessier, now recognized as the father of craniofacial surgery, first publicly presented his concepts of using wide exposure and a transcranial route to treating craniofacial deformities with large segmental movements of bone. An American disciple of Dr. Tessier, Dr. Linton Whitaker of the Children’s Hospital of Philadelphia, working with the Committee on Nomenclature and Classification of Craniofacial Anomalies of the American Cleft Palate-Craniofacial Association, presented a simple and practical classification system for craniofacial anomalies (Table 45-10).
It is the standard of care today that an interdisciplinary team of experts with specialized knowledge and training in treating children with craniofacial anomalies care for children who have such anomalies. The preoperative workup and evaluation must be thorough and should include imaging (computed tomography [CT], magnetic resonance imaging [MRI], and cephalography), photography, blood work, anesthesia consultation, and other components as the condition dictates. Craniofacial procedures are often long, complicated surgeries of significant magnitude, with an attendant risk of blood loss, serious morbidity, and even mortality. Significant blood loss is a realistic possibility, and preparation for blood conservation and transfusion must be made. The routine surgical approach to the craniofacial skeleton can be via a coronal incision, and after a bifrontal craniotomy, the orbital and facial skeleton can be addressed. Bone grafts for reconstruction can be split calvarial grafts or, alternatively, grafts from the ribs or iliac crest. Rigid fixation is obtained with bioresorbable plates, screws, and sutures. Despite the magnitude of the procedures, significant morbidity (e.g., blindness, brain injury, significant infection, cerebrospinal fluid leak, intracranial hematoma) or mortality is rare.
The rare craniofacial clefts have been subclassified by Tessier (Fig. 45-17). The Tessier classification of craniofacial clefts considers the orbit as the center around which the clefts radiate as the spokes of a wheel, numbered from 0 to 14. The facial clefts (0 to 7) and their cranial extensions (8 to 14) are often associated and total 14 (Fig. 45-18). Treacher Collins syndrome (Fig. 45-19), also known as mandibulofacial dysostosis, is a type of craniofacial clefting disorder representing bilateral 6-7-8 clefts. This autosomal dominant disorder with variable penetrance has the following manifestations: hypoplasia of the zygomas, asymmetry and hypoplasia of the mandible, ear anomalies, and colobomas of the lower eyelids. Craniofacial microsomia, also known as hemifacial microsomia, can be classified as a form of clefting as well (Fig. 45-20). Manifestations of this anomaly usually involve the hard and soft tissue of one half of the craniofacial skeleton. Deformities range in severity from complete absence of an affected facial component (globe, mandible, ear) to mild asymmetries. Ear deformities range from complete absence of the ear to only preauricular skin tags. Similarly, the eye deformities range from complete absence of the globe to various anomalies including epibulbar dermoids. Hypoplasia of the temporal skull, maxilla and zygoma, and orbit are seen in varying degree and affect the underlying skeleton as well as the overlying soft tissues. The classical deformity of hemifacial microsomia affects the mandible. Hypoplasia of the hemimandible, as well as the maxilla, results in dental malocclusions (Fig. 45-20C). Mandibular hypoplasia may range from minor underdevelopment of otherwise normal components to complete absence of the condyle, ramus, and proximal body.
Treatment of hemifacial microsomia includes management of the airway and attention to other functional conditions. Treatment of the mandibular deformity includes distraction osteogenesis during growth and orthognathic procedures at skeletal maturity. Ear deformities are reconstructed with techniques using costal cartilage and local soft tissue. Soft tissue deficiencies of the hemiface can be treated with fat injections, dermal-fat grafts, or free tissue transfer. Orbital hypertelorism is yet another type of midline craniofacial (0-14) clefting. Orbital hypertelorism is defined as a lateralization of the entire orbit, increasing the intraorbital distance and resulting from midline conditions such as encephaloceles, frontonasal dysplasia, and syndromic craniosynostosis. The treatment of severe orbital hypertelorism includes a transcranial approach to four-wall orbital box osteotomies, resection or treatment of the abnormal midline process, mobilization, medialization of the orbital complexes, and nasal reconstruction with a cantilever nasal bone graft.
The craniosynostoses are a group of disorders that result from the abnormal obliteration or premature fusion of the cranial sutures. The craniosynostoses can be subdivided into simple or single-suture craniosynostoses, and complex, syndromic, or multiple-suture craniosynostoses. The cranial sutures allow for the normal growth of the skull, and therefore, the classic presentation of craniosynostosis is an abnormal head shape. The resultant abnormal head shapes are secondary to an inhibition of skull growth at right angles to the fused suture and a compensatory overexpansion of the skull perpendicular to the fused suture into areas with open sutures. These abnormal head shapes provide a basis for the classification of craniosynostoses. In addition to appearance-related deformities resulting from craniosynostosis, important functional aspects include the potential for intracranial hypertension, which may result from brain growth restricted by an unyielding skull. The chances of intracranial hypertension increase with the number of sutures affected. Blindness and mental deficiencies secondary to an increase in intracranial pressure can likely be prevented by the surgical expansion of the cranium to release the fused suture, correct the abnormal head shape, and remodel the skull. The standard procedure used today in the correction of these synostotic deformities is fronto-orbital advancement. Fronto-orbital advancement, performed using a transcranial approach, includes a frontal craniotomy and orbital repositioning. The complex or multisutural synostoses are often syndromic, resulting from gain-of-function mutations of the fibroblast growth factor receptors (FGFR1, FGFR2, FGFR3). These syndromes of craniosynostosis include Apert’s, Crouzon’s, Pfeiffer’s, and Saethre-Chotzen syndromes. The syndromic craniosynostoses not only include bicoronal synostosis but also involve the midface, with resulting exorbitism and midface hypoplasia. Multilevel airway anomalies, obstructive sleep apnea, corneal exposure, intracranial hypertension, feeding difficulties, and severe malocclusion are some of the associated anomalies found in children with syndromic craniosynostoses. In addition to fronto-orbital advancement, facial osteotomies (i.e., Le Fort III craniofacial disjunction) are required to treat the orbital, midfacial, and occlusal deformities.
The categories of craniofacial atrophy and hypoplasia encompass many conditions such as Pierre Robin sequence and Romberg’s progressive hemifacial atrophy. Pierre Robin sequence is characterized by three pathognomonic findings: microretrognathia, glossoptosis, and respiratory distress. Pierre Robin sequence may or may not be associated with a palatal cleft. It is thought by some to occur secondary to a fixed and flexed fetal head position that inhibits mandibular growth and results in micrognathia. The micrognathia prevents the natural caudal migration of the tongue from between the clefted palatal shelves, and the resulting deformity as described earlier. The functional consequences include intermittent respiratory obstruction and obstructive sleep apnea that may affect feeding, growth, and safety of the airway. Treatment of a child mildly affected with Pierre Robin sequence may include simply positioning the child prone until the child “grows out” of the condition. However, if the child is severely affected and unable to feed adequately or has an unsafe airway, surgical intervention is required. For decades, tracheotomy was the initial and definitive treatment of choice; however, today many initially attempt a tongue-lip adhesion, treating the glossoptosis and alleviating respiratory obstruction by suturing the tongue tip to the lower lip. The tongue-lip adhesion is taken down at the time of palatoplasty. Should the tongue-lip adhesion not adequately correct the obstruction, then neonatal mandibular distraction can be used to correct the underlying microretrognathia and relieve the obstructive symptoms (Fig. 45-21). Another syndrome of atrophy and hypoplasia is Romberg’s progressive hemifacial atrophy, also known as Parry-Romberg syndrome (Fig. 45-22). Romberg’s disease is a disorder of unknown etiology, beginning in childhood or adolescence, in which hemifacial atrophy of the skin, subcutaneous fat, muscle, bone, and cartilage progresses for a variable period of time before spontaneously ceasing or “burning out” 2 to 10 years after beginning. Most believe treatment should be delayed until at least 1 year after the process of atrophy has ceased. Some hematologists and oncologists have treated the early presentation of Romberg’s disease with chemotherapy. After the cessation of atrophy, reconstruction of the craniofacial skeleton and soft tissues may begin with bone and/or cartilage grafts, alloplastic implants, dermal-fat grafts, fat grafting, and possibly free tissue transfers.
Figure 45-21.
A. Lateral view of a child with Pierre Robin sequence and mandibular microretrognathia. B. Intraoperative photo of a submandibular incision and planning for the placement of a buried mandibular distractor. C. Lateral view of the child after mandibular distraction with slight overcorrection of retrognathia. The distractor is still in place as evident from the activating rod seen exiting the skin retroauricularly.
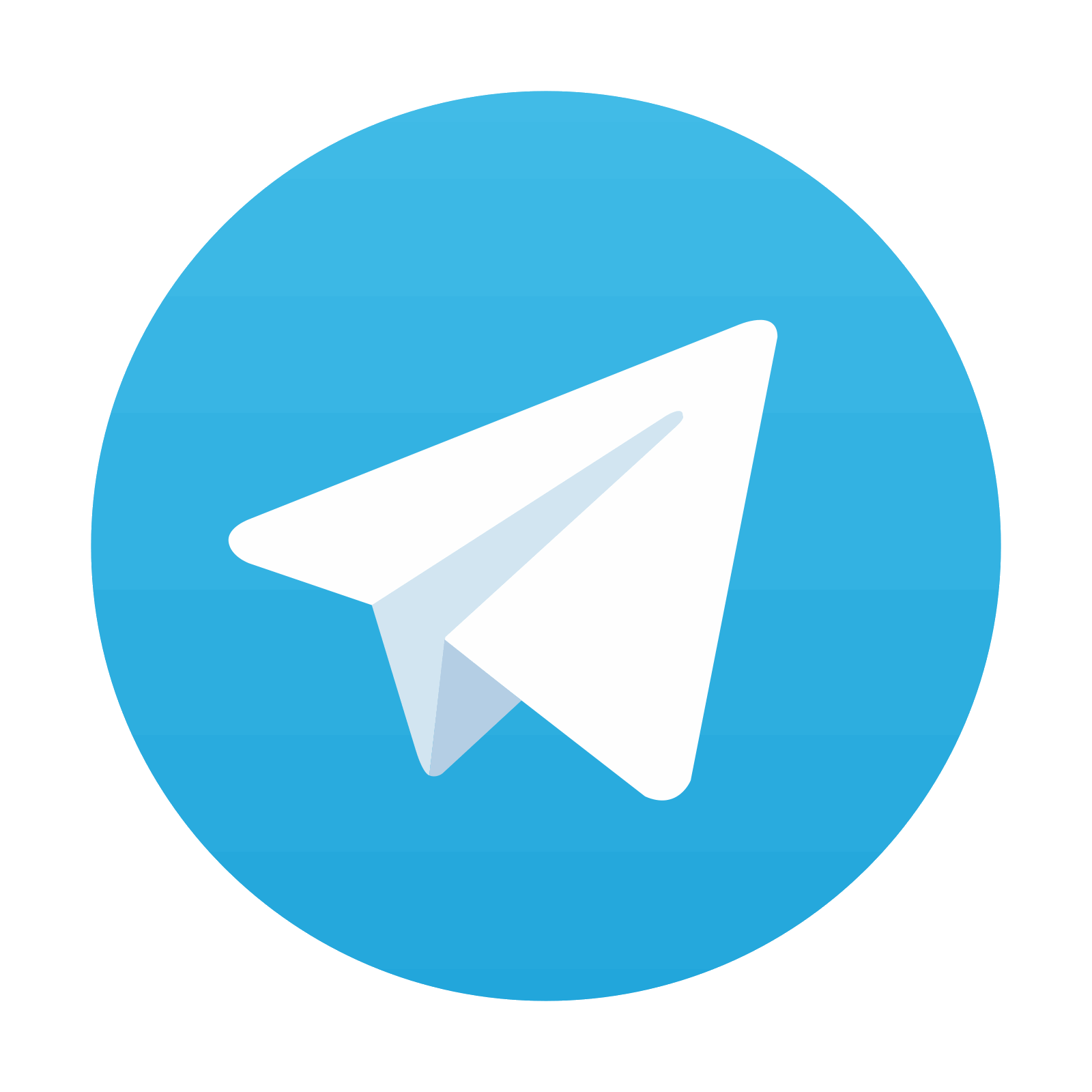
Stay updated, free articles. Join our Telegram channel
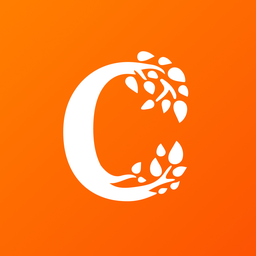
Full access? Get Clinical Tree
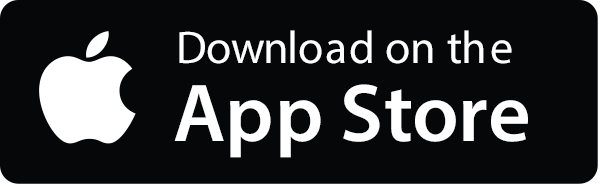
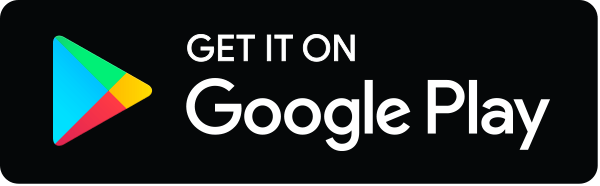