KEY POINTS
The delivery of modern critical care is predicated on the ability to monitor a large number of physiologic variables and formulate evidenced-based therapeutic strategies to manage these variables. Technological advances in monitoring have at least a theoretical risk of exceeding our ability to understand the clinical implications of the derived information. This could result in the use of monitoring data to make inappropriate clinical decisions. Therefore, the implementation of any new monitoring technology must take into account the relevance and accuracy of the data obtained, the risks to the patient, as well as the evidence supporting any intervention directed at correcting the detected abnormality.
The routine use of invasive monitoring devices, specifically the pulmonary artery catheter, must be questioned in light of the available evidence which does not demonstrate a clear benefit to its widespread use in various populations of critically ill patients. The future of physiologic monitoring will be dominated by the application of noninvasive and highly accurate devices which guide evidenced-based therapy.
INTRODUCTION
The Latin verb monere, which means “to warn, or advise” is the origin for the English word monitor. In modern medical practice, patients undergo monitoring to detect pathologic variations in physiologic parameters, providing advanced warning of impending deterioration in the status of one or more organ systems. The intended goal of this endeavor is to allow the clinician to take appropriate actions in a timely fashion to prevent or ameliorate the physiologic derangement. Furthermore, physiologic monitoring is used not only to warn, but also to titrate therapeutic interventions, such as fluid resuscitation or the infusion of vasoactive or inotropic drugs. The intensive care unit (ICU) and operating room are the two locations where the most advanced monitoring capabilities routinely are employed in the care of critically ill patients.
In the broadest sense, physiologic monitoring encompasses a spectrum of endeavors, ranging in complexity from the routine and intermittent measurement of the classic vital signs (i.e., temperature, heart rate, arterial blood pressure, and respiratory rate) to the continuous recording of the oxidation state of cytochrome oxidase, the terminal element in the mitochondrial electron transport chain. The ability to assess clinically relevant parameters of tissue and organ status and employ this knowledge to improve patient outcomes represents the “holy grail” of critical care medicine. Unfortunately, consensus often is lacking regarding the most appropriate parameters to monitor in order to achieve this goal. Furthermore, making an inappropriate therapeutic decision due to inaccurate physiologic data or misinterpretation of good data can lead to a worse outcome than having no data at all. Of the highest importance is the integration of physiologic data obtained from monitoring into a coherent and evidenced-based treatment plan. Current technologies available to assist the clinician in this endeavor are summarized in this chapter. Also presented is a brief look at emerging techniques that may soon enter into clinical practice.
In essence, the goal of hemodynamic monitoring is to ensure that the flow of oxygenated blood through the microcirculation is sufficient to support aerobic metabolism at the cellular level. In general, mammalian cells cannot store oxygen for subsequent use in oxidative metabolism, although a relatively tiny amount is stored in muscle tissue as oxidized myoglobin. Thus, aerobic synthesis of adenosine triphosphate (ATP), the energy “currency” of cells, requires the continuous delivery of oxygen by diffusion from hemoglobin in red blood cells to the oxidative machinery within mitochondria. Delivery of oxygen to mitochondria may be insufficient for several reasons. For example, cardiac output, hemoglobin concentration of blood, or the oxygen content of arterial blood each can be inadequate for independent reasons. Alternatively, despite adequate cardiac output, perfusion of capillary networks can be impaired as a consequence of dysregulation of arteriolar tone, microvascular thrombosis, or obstruction of nutritive vessels by sequestered leukocytes or platelets. Hemodynamic monitoring that does not take into account all of these factors will portray an incomplete and perhaps misleading picture of cellular physiology.
Under normal conditions when the supply of oxygen is plentiful, aerobic metabolism is determined by factors other than the availability of oxygen. These factors include the hormonal milieu and mechanical workload of contractile tissues. However, in pathologic circumstances when oxygen availability is inadequate, oxygen utilization (VO2) becomes dependent upon oxygen delivery (DO2). The relationship of VO2 to DO2 over a broad range of DO2 values is commonly represented as two intersecting straight lines. In the region of higher DO2 values, the slope of the line is approximately zero, indicating that VO2 is largely independent of DO2. In contrast, in the region of low DO2 values, the slope of the line is nonzero and positive, indicating that VO2 is supplydependent. The region where the two lines intersect is called the point of critical oxygen delivery (DO2crit), and represents the transition from supply-independent to supply-dependent oxygen uptake. Below a critical threshold of oxygen delivery, increased oxygen extraction cannot compensate for the delivery deficit; hence, oxygen consumption begins to decrease. The slope of the supply-dependent region of the plot reflects the maximal oxygen extraction capability of the vascular bed being evaluated.
The subsequent sections will describe the techniques and utility of monitoring various physiologic parameters.
The pressure exerted by blood in the systemic arterial system, commonly referred to as “blood pressure,” is a cardinal parameter measured as part of the hemodynamic monitoring of patients. Extremes in blood pressure are either intrinsically deleterious or are indicative of a serious perturbation in normal physiology. Arterial blood pressure is a complex function of both cardiac output and vascular input impedance. Thus, inexperienced clinicians may assume that the presence of a normal blood pressure is evidence that cardiac output and tissue perfusion are adequate. This assumption frequently is incorrect and is the reason why some critically ill patients may benefit from forms of hemodynamic monitoring in addition to measurement of arterial pressure.
Blood pressure can be determined directly by measuring the pressure within the arterial lumen or indirectly using a cuff around an extremity. When the equipment is properly set up and calibrated, direct intra-arterial monitoring of blood pressure provides accurate and continuous data. Additionally, intra-arterial catheters provide a convenient way to obtain samples of blood for measurements of arterial blood gases and other laboratory studies. Despite these advantages, intra-arterial catheters are invasive devices and occasionally are associated with serious complications.
Both manual and automated means for the noninvasive determination of blood pressure use an inflatable sphygmomanometer cuff to increase pressure around an extremity, and a means for detecting the presence or absence of arterial pulsations. Several methods exist for this purpose. The time-honored approach is the auscultation of the Korotkoff sounds, which are heard over an artery distal to the cuff as the cuff is deflated from a pressure higher than systolic pressure to one less than diastolic pressure. Systolic pressure is defined as the pressure in the cuff when tapping sounds are first audible. Diastolic pressure is the pressure in the cuff when audible pulsations first disappear.
Another means for pulse detection when measuring blood pressure noninvasively depends upon the detection of oscillations in the pressure within the bladder of the cuff. This approach is simple, and unlike auscultation, can be performed even in a noisy environment (e.g., a busy emergency room). Unfortunately, this approach is neither accurate nor reliable. Other methods, however, can be used to reliably detect the reappearance of a pulse distal to the cuff and thereby estimate systolic blood pressure. Two excellent and widely available approaches for pulse detection are use of a Doppler stethoscope (reappearance of the pulse produces an audible amplified signal) or a pulse oximeter (reappearance of the pulse is indicated by flashing of a light-emitting diode).
A number of automated devices are capable of repetitively measuring blood pressure noninvasively. Some of these devices measure pressure oscillations in the inflatable bladder encircling the extremity to detect arterial pulsations as pressure in the cuff is gradually lowered from greater than systolic to less than diastolic pressure. Other automated noninvasive devices use a piezoelectric crystal positioned over the brachial artery as a pulse detector. The accuracy of these devices is variable, and often dependent on the size mismatch between the arm circumference and the cuff size.1 If the cuff is too narrow (relative to the extremity), the measured pressure will be artifactually elevated. Therefore, the width of the cuff should be approximately 40% of its circumference.
Another noninvasive approach for measuring blood pressure relies on a technique called photoplethysmography. This method is capable of providing continuous information, since systolic and diastolic blood pressures are recorded on a beat-to-beat basis. Photoplethysmography uses the transmission of infrared light to estimate the amount of hemoglobin (directly related to the volume of blood) in a finger placed under a servo-controlled inflatable cuff. A feedback loop controlled by a microprocessor continually adjusts the pressure in the cuff to maintain the blood volume of the finger constant. Under these conditions, the pressure in the cuff reflects the pressure in the digital artery. The measurements obtained using photoplethysmography generally agree closely with those obtained by invasive monitoring of blood pressure.2 However, these readings may be less accurate in patients with hypotension or hypothermia.
Direct and continuous monitoring of arterial pressure in critically ill patients may be performed by using fluid-filled tubing to connect an intra-arterial catheter to an external strain-gauge transducer. The signal generated by the transducer is electronically amplified and displayed as a continuous waveform by an oscilloscope. Digital values for systolic and diastolic pressure also are displayed. Mean pressure, calculated by electronically averaging the amplitude of the pressure waveform, also can be displayed. The fidelity of the catheter-tubing-transducer system is determined by numerous factors, including the compliance of the tubing, the surface area of the transducer diaphragm, and the compliance of the diaphragm. If the system is underdamped, then the inertia of the system, which is a function of the mass of the fluid in the tubing and the mass of the diaphragm, causes overshoot of the points of maximum positive and negative displacement of the diaphragm during systole and diastole, respectively. Thus, in an underdamped system, systolic pressure will be overestimated and diastolic pressure will be underestimated. In an overdamped system, displacement of the diaphragm fails to track the rapidly changing pressure waveform, and systolic pressure will be underestimated and diastolic pressure will be overestimated. It is important to note that even in an underdamped or over-damped system, mean pressure will be accurately recorded, provided the system has been properly calibrated. For these reasons, when using direct measurement of intra-arterial pressure to monitor patients, clinicians should make clinical decisions based primarily on the measured mean arterial blood pressure.
The radial artery at the wrist is the site most commonly used for intra-arterial pressure monitoring. Other sites include the femoral and axillary artery. It is important to recognize, however, that measured arterial pressure is determined in part by the site where the pressure is monitored. Central (i.e., aortic) and peripheral (e.g., radial artery) pressures typically are different as a result of the impedance and inductance of the arterial tree. Systolic pressures typically are higher and diastolic pressures are lower in the periphery, whereas mean pressure is approximately the same in the aorta and more distal sites.
Distal ischemia is an uncommon complication of intra-arterial catheterization. The incidence of thrombosis is increased when larger-caliber catheters are employed and when catheters are left in place for an extended period of time. The incidence of thrombosis can be minimized by using a 20-gauge (or smaller) catheter in the radial artery and removing the catheter as soon as feasible. The risk of distal ischemic injury can be reduced by ensuring that adequate collateral flow is present prior to catheter insertion. At the wrist, adequate collateral flow can be documented by performing a modified version of the Allen test, wherein the artery to be cannulated is digitally compressed while using a Doppler stethoscope to listen for perfusion in the palmar arch vessels.
Another potential complication of intra-arterial monitoring is retrograde embolization of air bubbles or thrombi into the intracranial circulation. In order to minimize this risk, care should be taken to avoid flushing arterial lines when air is present in the system, and only small volumes of fluid (less than 5 mL) should be employed for this purpose. Catheter-related infections can occur with any intravascular monitoring device. However, catheter-related bloodstream infection is a relatively uncommon complication of intra-arterial lines used for monitoring, occurring in 0.4% to 0.7% of catheterizations.3 The incidence increases with longer duration of arterial catheterization.
The electrocardiogram (ECG) records the electrical activity associated with cardiac contraction by detecting voltages on the body surface. A standard 3-lead ECG is obtained by placing electrodes that correspond to the left arm (LA), right arm (RA), and left leg (LL). The limb leads are defined as lead I (LA-RA), lead II (LL-RA), and lead III (LL-LA). The ECG waveforms can be continuously displayed on a monitor, and the devices can be set to sound an alarm if an abnormality of rate or rhythm is detected. Continuous ECG monitoring is widely available and applied to critically ill and perioperative patients. Monitoring of the ECG waveform is essential in patients with acute coronary syndromes or blunt myocardial injury, because dysrhythmias are the most common lethal complication. In patients with shock or sepsis, dysrhythmias can occur as a consequence of inadequate myocardial oxygen delivery or as a complication of vasoactive or inotropic drugs used to support blood pressure and cardiac output. Dysrhythmias can be detected by continuously monitoring the ECG tracing, and timely intervention may prevent serious complications. With appropriate computing hardware and software, continuous ST-segment analysis also can be performed to detect ischemia or infarction.
Additional information can be obtained from a 12-lead ECG, which is essential for patients with potential myocardial ischemia or to rule out cardiac complications in other acutely ill patients. Continuous monitoring of the 12-lead ECG is now available and is proving to be beneficial in certain patient populations. In a study of 185 vascular surgical patients, continuous 12-lead ECG monitoring was able to detect transient myocardial ischemic episodes in 20.5% of the patients.4 This study demonstrated that the precordial lead V4, which is not routinely monitored on a standard 3-lead ECG, is the most sensitive for detecting perioperative ischemia and infarction. To detect 95% of the ischemic episodes, two or more precordial leads were necessary. Thus, continuous 12-lead ECG monitoring may provide greater sensitivity than 3-lead ECG for the detection of perioperative myocardial ischemia, and may become standard for monitoring high-risk surgical patients.
Currently, there is considerable interest in using computerized approaches to analyze ECG waveforms and patterns to uncover hidden information that can be used to predict sudden cardiac death or the development of serious dysrhythmias. ECG patterns of interest include repetitive changes in the morphology of the T-wave [T-wave alternans (TWA)]5and heart rate variability.6
Integrated monitoring systems employ software that integrates vital signs to produce a single-parameter index which allows early detection of physiologic perturbations. The input variables include noninvasive measurements of heart rate, respiratory rate, blood pressure, blood oxygen saturation via pulse oximetry (SpO2), and temperature. The software uses sophisticated algorithms refined in an iterative fashion to develop a probabilistic model of normality, previously developed from a representative sample patient training set. Variance from these data set are used to evaluate the probability that the patient-derived vital signs are within the normal range. An abnormal index can occur while no single vital sign parameter is outside the range of normal if their combined patterns are consistent with known instability patterns. Employing such an integrated monitoring system in step-down unit patients has been shown to be a sensitive method to detect early physiologic abnormalities that may precede hemodynamic instability.7
Bedside catheterization of the pulmonary artery was introduced into clinical practice in the 1970s. Although the pulmonary artery catheter (PAC) initially was used primarily to manage patients with cardiogenic shock and other acute cardiac diseases, indications for this form of invasive hemodynamic monitoring gradually expanded to encompass a wide variety of clinical conditions. Clearly, many clinicians believe that information valuable for the management of critically ill patients is afforded by having a PAC in place. However, unambiguous data in support of this view are scarce, and several studies suggest that bedside PAC may not benefit most critically ill patients, and in fact lead to some serious complications (see next).
Starling’s law of the heart states that the force of muscle contraction depends on the initial length of the cardiac fibers. Using terminology that derives from early experiments using isolated cardiac muscle preparations, preload is the stretch of ventricular myocardial tissue just prior to the next contraction. Thus, cardiac preload is determined by end-diastolic volume (EDV). For the right ventricle, central venous pressure (CVP) approximates right ventricular end-diastolic pressure (EDP). For the left ventricle, pulmonary artery occlusion pressure (PAOP), which is measured by transiently inflating a balloon at the end of a pressure monitoring catheter positioned in a small branch of the pulmonary artery, approximates left ventricular end-diastolic pressure. The presence of atrioventricular valvular stenosis may alter this relationship.
Clinicians frequently use EDP as a surrogate for EDV, but EDP is determined not only by volume but also by the diastolic compliance of the ventricular chamber. Ventricular compliance is altered by various pathologic conditions and pharmacologic agents. Furthermore, the relationship between EDP and true preload is not linear, but rather is exponential.
Afterload is another term derived from in vitro experiments using isolated strips of cardiac muscle, and is defined as the force resisting fiber shortening once systole begins. Several factors comprise the in vivo correlate of ventricular afterload, including ventricular intracavitary pressure, wall thickness, chamber radius, and chamber geometry. Since these factors are difficult to assess clinically, afterload is commonly approximated by calculating systemic vascular resistance, defined as mean arterial pressure (MAP) divided by cardiac output.
Contractility is defined as the inotropic state of the myocardium. Contractility is said to increase when the force of ventricular contraction increases at constant preload and afterload. Clinically, contractility is difficult to quantify, because virtually all of the available measures are dependent to a certain degree on preload and afterload. If pressure-volume loops are constructed for each cardiac cycle, small changes in preload and/or afterload will result in shifts of the point defining the end of systole. These end-systolic points on the pressure vs. volume diagram describe a straight line, known as the end-systolic pressure-volume line. A steeper slope of this line indicates greater contractility.
In its simplest form, the pulmonary artery catheter (PAC) has four channels. One channel terminates in a balloon at the tip of the catheter. The proximal end of this channel is connected to a syringe to permit inflation of the balloon with air (saline should never be used). Prior to insertion of the PAC, the integrity of the balloon should be verified by inflating it. In order to minimize the risk of vascular or ventricular perforation by the relatively inflexible catheter, it also is important to verify that the inflated balloon extends just beyond the tip of the device. A second channel in the catheter contains wires that are connected to a thermistor located near the tip of the catheter. At the proximal end of the PAC, the wires terminate in a fitting that permits connection to appropriate hardware for the calculation of cardiac output using the thermodilution technique (see next). The final two channels are used for pressure monitoring and the injection of the thermal indicator for determinations of cardiac output. One of these channels terminates at the tip of the catheter. The other terminates 20 cm proximal to the tip.
Placement of a PAC requires access to the central venous circulation. Such access can be obtained at a variety of sites, including the antecubital, femoral, jugular, and subclavian veins. Percutaneous placement through either the jugular or subclavian vein generally is preferred. Right internal jugular vein cannulation carries the lowest risk of complications, and the path of the catheter from this site into the right atrium is straight. In the event of inadvertent arterial puncture, local pressure is significantly more effective in controlling bleeding from the carotid artery compared to the subclavian artery. Nevertheless, it is more difficult to keep occlusive dressings in place on the neck than in the subclavian fossa. Furthermore, the anatomic landmarks in the subclavian position are quite constant, even in patients with anasarca or massive obesity; the subclavian vein always is attached to the deep (concave) surface of the clavicle. In contrast, the appropriate landmarks to guide jugular venous cannulation are sometimes difficult to discern in obese or very edematous patients. However, ultrasonic guidance, which should be used routinely, has been shown to facilitate bedside jugular venipuncture.8
Cannulation of the vein normally is performed percutaneously, using the Seldinger technique. A small-bore needle is inserted through the skin and subcutaneous tissue into the vein. After documenting return of venous blood, a guidewire with a flexible tip is inserted through the needle into the vein and the needle is withdrawn. A dilator/introducer sheath is passed over the wire, and the wire and the dilator are removed. The proximal terminus of the distal port of the PAC is connected through low-compliance tubing to a strain-gauge transducer, and the tubing-catheter system is flushed with fluid. While constantly observing the pressure tracing on an oscilloscope, the PAC is advanced with the balloon deflated until respiratory excursions are observed. The balloon is then inflated, and the catheter advanced further (“floated”), while monitoring pressures sequentially in the right atrium and right ventricle en route to the pulmonary artery. The pressure waveforms for the right atrium, right ventricle, and pulmonary artery are each characteristic. The catheter is advanced out into the pulmonary artery until a damped tracing indicative of the “wedged” position is obtained. The balloon is then deflated, taking care to ensure that a normal pulmonary arterial tracing is again observed on the monitor; leaving the balloon inflated can increase the risk of pulmonary infarction or perforation of the pulmonary artery. Unnecessary measurements of the pulmonary artery occlusion pressure are discouraged as rupture of the pulmonary artery may occur.
Even in its simplest embodiment, the PAC is capable of providing clinicians with a remarkable amount of information about the hemodynamic status of patients. Additional information may be obtained if various modifications of the standard PAC are employed. By combining data obtained through use of the PAC with results obtained by other means (i.e., blood hemoglobin concentration and oxyhemoglobin saturation), derived estimates of systemic oxygen transport and utilization can be calculated. Direct and derived parameters obtainable by bedside pulmonary arterial catheterization are summarized in Table 13-1. The equations used to calculate the derived parameters are summarized in Table 13-2. The approximate normal ranges for a number of these hemodynamic parameters (in adults) are shown in Table 13-3.
STANDARD PAC | PAC WITH ADDITIONAL FEATURE(S) | DERIVED PARAMETERS |
---|---|---|
CVP | S⊽o2 (continuous) | SV (or SVI) |
PAP | QT or QT* (continuous) | SVR (or SVRI) |
PAOP | RVEF | PVR (or PVRI) |
S⊽o2 (intermittent) | RVEDV | |
QT or QT* (intermittent) | Ḋo2 ⊽o2 ER QS/QT |
QT* (L·min–1·m–2) = QT/BSA, where BSA is body surface area (m2) SV (mL) = QT/HR, where HR is heart rate (min–1) SVR (dyne·sec·cm–5) = [(MAP – CVP) × 80] /QT, where MAP is mean arterial pressure (mm Hg) SVRI (dyne·sec·cm–5m–2) = [(MAP – CVP) × 80] /QT* PVR (dyne·sec·cm–5) = [(PAP – PAOP) × 80] /QT, where PAP is mean pulmonary artery pressure PVRI (dyne·sec·cm–5m–2) = [(PAP – PAOP) × 80] /QT* RVEDV (mL) = SV/RVEF Ḋo2(mL·min–1·m–2) = QT* × Cao2 × 10, where Cao2 is arterial oxygen content (mL/dL) ⊽o2(mL·min–1·m–2) = QT* × (Cao2 – C⊽o2) × 10, where C⊽o2 is mixed venous oxygen content (mL/dL) Cao2 = (1.36 × Hgb × Sao2) + (0.003 + Pao2), where Hgb is hemoglobin concentration (g/dL), Sao2 is fractional arterial hemoglobin saturation, and Pao2 is the partial pressure of oxygen in arterial blood C⊽o2 = (1.36 × Hgb × S⊽o2) + (0.003 + P⊽o2), where P ⊽o2 is the partial pressure of oxygen in pulmonary arterial (mixed venous) blood QS/QT = (Cco2 – Ca o2)/ (Cco2 – Cv o2), where Cc o2 (mL/dL) is the content of oxygen in pulmonary end capillary blood Cco2 = (1.36 × Hgb) + (0.003 + Pao2), where Pao2 is the alveolar partial pressure of oxygen Pao2 = [Fio2 × (PB – PH2O)] – Paco2/RQ, where Fio2 is the fractional concentration of inspired oxygen, PB is the barometric pressure (mm Hg), PH2O is the water vapor pressure (usually 47 mm Hg), Paco2 is the partial pressure of carbon dioxide in arterial blood (mm Hg), and RQ is respiratory quotient (usually assumed to be 0.8) |
PARAMETER | NORMAL RANGE |
---|---|
CVP | 0–6 mm Hg |
Right ventricular systolic pressure | 20–30 mm Hg |
Right ventricular diastolic pressure | 0–6 mm Hg |
PAOP | 6–12 mm Hg |
Systolic arterial pressure | 100–130 mm Hg |
Diastolic arterial pressure | 60–90 mm Hg |
MAP | 75–100 mm Hg |
QT | 4–6 L/min |
QT* | 2.5–3.5 L·min–1·m–2 |
SV | 40–80 mL |
SVR | 800–1400 dyne·sec·cm–5 |
SVRI | 1500–2400 dyne·sec·cm–5·m–2 |
PVR | 100–150 dyne·sec·cm–5 |
PVRI | 200–400 dyne·sec·cm–5·m–2 |
Cao2 | 16–22 mL/dL |
Cvo2 | ~15 mL 02 dL blood |
Ḋo2 | 400–660 mL·min–1·m–2 |
⊽o2 | 115–165 mL·min–1·m–2 |
Before the development of the PAC, determining cardiac output (QT) at the bedside required careful measurements of oxygen consumption (Fick method) or spectrophotometric determination of indocyanine green dye dilution curves. Measurements of QT using the thermodilution technique are simple and reasonably accurate. The measurements can be performed repetitively and the principle is straightforward. If a bolus of an indicator is rapidly and thoroughly mixed with a moving fluid upstream from a detector, then the concentration of the indicator at the detector will increase sharply and then exponentially diminish back to zero. The area under the resulting time-concentration curve is a function of the volume of indicator injected and the flow rate of the moving stream of fluid. Larger volumes of indicator result in greater areas under the curve, and faster flow rates of the mixing fluid result in smaller areas under the curve. When QT is measured by thermodilution, the indicator is heat and the detector is a temperature-sensing thermistor at the distal end of the PAC. The relationship used for calculating QT is called the Stewart-Hamilton equation:
where V is the volume of the indicator injected, TB is the temperature of blood (i.e., core body temperature), TI is the temperature of the indicator, K1 is a constant that is the function of the specific heats of blood and the indicator, K2 is an empirically derived constant that accounts for several factors (the dead space volume of the catheter, heat lost from the indicator as it traverses the catheter, and the injection rate of the indicator), and ∫TB(t)dt is the area under the time-temperature curve. In clinical practice, the Stewart-Hamilton equation is solved by a microprocessor.
Determination of cardiac output by the thermodilution method is generally quite accurate, although it tends to systematically overestimate QT at low values. Changes in blood temperature and QT during the respiratory cycle can influence the measurement. Therefore, results generally should be recorded as the mean of two or three determinations obtained at random points in the respiratory cycle. Using cold injectate widens the difference between TB and TI and thereby increases signal-to-noise ratio. Nevertheless, most authorities recommend using room temperature injectate (normal saline or 5% dextrose in water) to minimize errors resulting from warming of the fluid as it transferred from its reservoir to a syringe for injection.
Technologic innovations have been introduced that permit continuous measurement of QT by thermodilution. In this approach, thermal transients are not generated by injecting a bolus of a cold indicator, but rather by heating the blood with a tiny filament located on the PAC upstream from the thermistor. By correlating the amount of current supplied to the heating element with the downstream temperature of the blood, it is possible to estimate the average blood flow across the filament and thereby calculate QT. Based upon the results of several studies, continuous determinations of QT using this approach agree well with data generated by conventional measurements using bolus injections of a cold indicator.9 Information is lacking regarding the clinical value of being able to monitor QT continuously.
The Fick equation can be written as QT = VO2/(Cao2 – CVO2), where Cao2 is the content of oxygen in arterial blood and CVO2 is the content of oxygen in mixed venous blood. The Fick equation can be rearranged as follows: CVO2 = Cao2 – VO2/QT. If the small contribution of dissolved oxygen to CVO2 and Cao2 is ignored, the rearranged equation can be rewritten as SVO2 = Sao2 – VO2/(QT × Hgb × 1.36), where SVO2 is the fractional saturation of hemoglobin in mixed venous blood, Sao2 is the fractional saturation of hemoglobin in arterial blood, and Hgb is the concentration of hemoglobin in blood. Thus it can be seen that SVO2 is a function of VO2 (i.e., metabolic rate), QT, Sao2, and Hgb. Accordingly, subnormal values of SVO2 can be caused by a decrease in QT (due, for example, to heart failure or hypovolemia), a decrease in Sao2 (due, for example, to intrinsic pulmonary disease), a decrease in Hgb (i.e., anemia), or an increase in metabolic rate (due, for example, to seizures or fever). With a conventional PAC, measurements of SVO2 require aspirating a sample of blood from the distal (i.e., pulmonary arterial) port of the catheter and injecting the sample into a blood gas analyzer. Therefore for practical purposes, measurements of SVO2 can be performed only intermittently.
By adding a fifth channel to the PAC, it has become possible to monitor SVO2
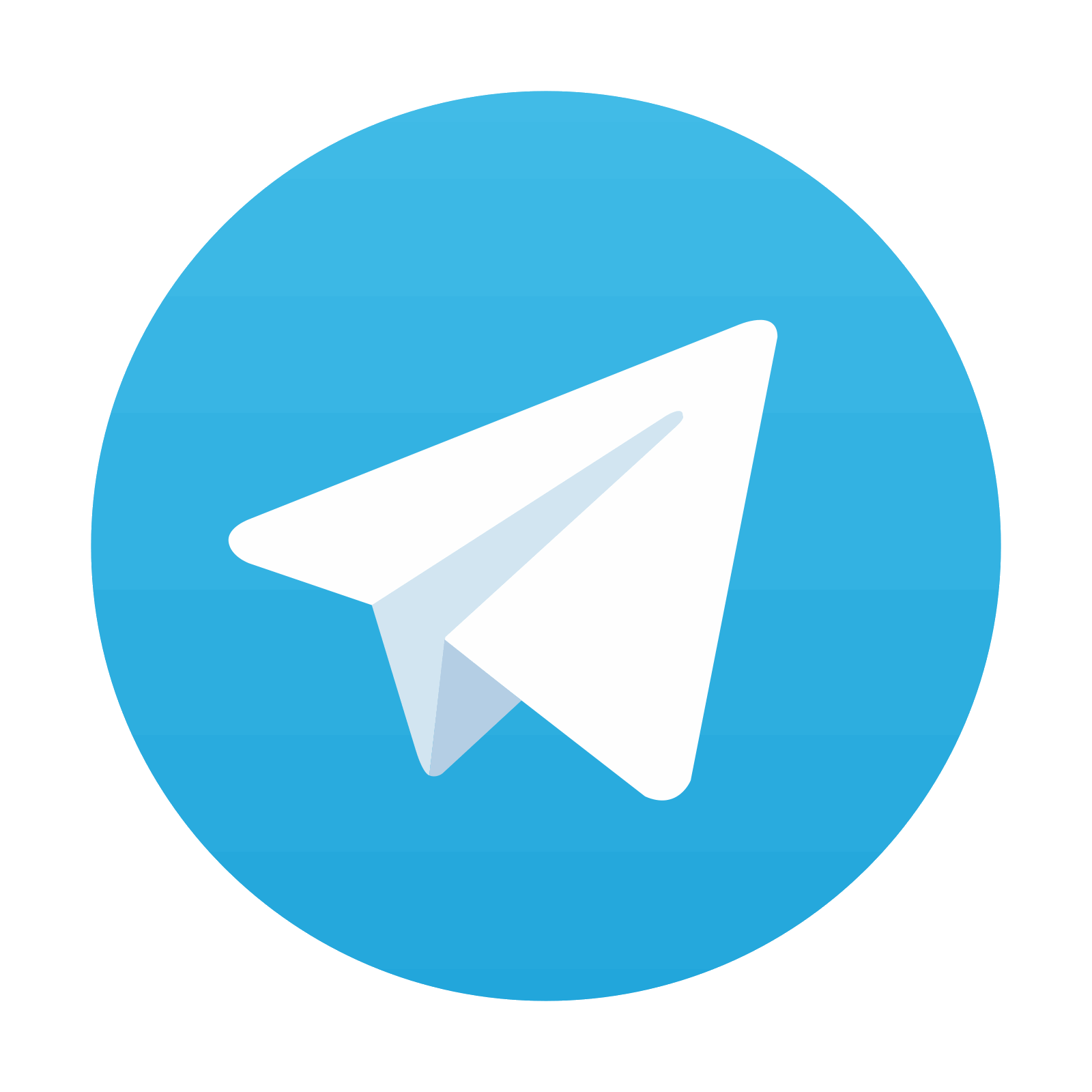
Stay updated, free articles. Join our Telegram channel
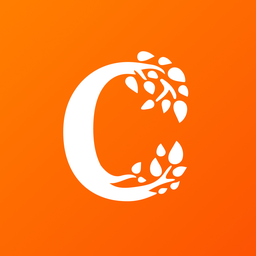
Full access? Get Clinical Tree
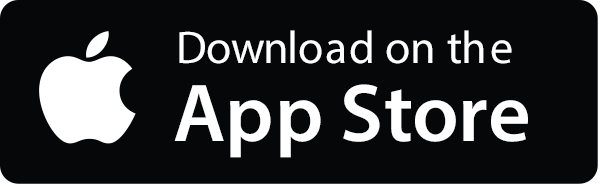
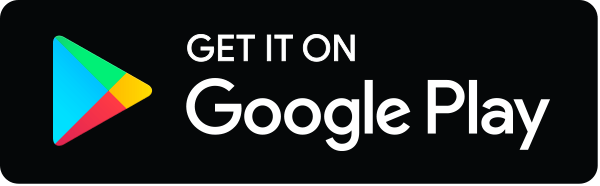
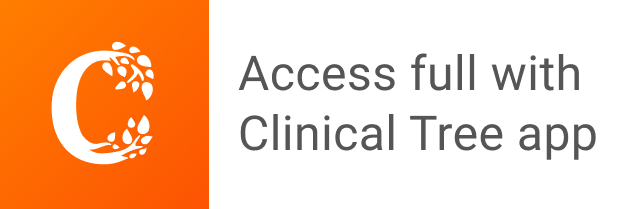