Organ Pathogenesis: Lung, Heart, Kidney, Liver, Pancreas, Brain
Nagme Bilgehan
Galatea Stavraka
Justin Stebbing
INTRODUCTION
During the early stages of the coronavirus disease 2019 (COVID-19) outbreak in 2020, the general consensus was that the virus predominantly targeted lung function, as the most common symptoms patients presented with, if any, involved the respiratory system. However, in due course, varying clinical presentations started to arise, or rather we became more aware of them, highlighting additional features of the virus’s pathogenicity, in turn distinguishing it from other respiratory viruses, notably influenza. In some cases, patients would present with multiorgan failure, and other severe extrapulmonary complications including, but not limited to, heart disease and neurologic disorders.1 With the emergence of these new presentations, the scientific community was able to conduct further research that highlighted the complex nature of the disease.
Parts of the disease’s pathogenesis can be understood by looking at the mechanism by which it invades host cells. Severe acute respiratory syndrome coronavirus 2 (SARS-CoV-2) is comprised of four structural proteins, spike (S), membrane (M), envelope (E), and nucleocapsid (N). Spike is comprised of two subunits, S1, which binds to the angiotensin-converting enzyme 2 (ACE2) receptor on host cells, and S2, which aids membrane fusion. After the S1 subunit binds to ACE2, transmembrane serine protease 2 (TMPRSS2) cleaves the spike protein at S2, activating this domain to fuse the viral and host membranes for entry of the virus into the cell.2,3 Binding of the virus to ACE2 causes downregulation of ACE2 surface expression. ACE2 inhibits the renin-angiotensin-aldosterone system (RAAS) by degrading angiotensin II, a vasoconstrictive agent that increases blood pressure, into angiotensin 1-7, which causes vasodilation and lowers blood pressure.4 It is expressed in various parts of the body, abundantly in the lung, heart, kidney, and intestines, which is important for both viral tropism and its use as a potential therapeutic target in COVID-19.2
Although various aspects of SARS-CoV-2 pathogenesis are related to the direct effects it has on cells and organs, a significant part is related to the dysfunction of the immune system because of the virus. After cells are infected by the virus, the subsequent cell injury or necrosis triggers the release of damage-associated molecular patterns (DAMPs), which signal innate immune cells such as macrophages and neutrophils to release a variety of proinflammatory cytokines, chemokines, and antiviral molecules to combat the infection.5 Immune homeostasis can be disrupted because of COVID-19 infection, which when paired with an influx of hyperinflammatory immune cells may result in localized or systemic tissue damage affecting a multitude of organs, namely the lungs, heart, kidneys, liver, pancreas, and brain (Figure 10.1).
THE LUNGS
Although multiple organs can be affected by the virus, the lungs are predominantly affected, with the subsequent lung damage being the leading cause of COVID-19 deaths.6 The most common respiratory symptoms experienced by patients include dyspnea, a dry cough, a sore throat, and rhinorrhea. Interestingly, a phenomenon referred to as “silent hypoxemia” can be seen in patients with COVID-19, where there is a critically low PaO2 but mild dyspnea or respiratory discomfort.7
Radiologically, SARS-CoV-2 does seem to have some distinguishing features from other viruses and can present with peripheral opacification, glass opacities, and vascular thickening. As the disease progresses and becomes more severe, the lesions tend to present bilaterally, more abundant in the lower lung, and can have a rounded appearance, termed “COVID balls.”8 A study investigating radiologic findings in patients with COVID-19 in Wuhan demonstrated that on chest computed tomography (CT), COVID-19 pneumonia can manifest with rapid evolution from focal unilateral to diffuse bilateral ground-glass opacities, which can progress to consolidations within 1 to 3 weeks. They also noted that chest CT abnormalities can be present even in asymptomatic patients.9
Because of factors including but not limited to preexisting chronic lung problems, there is a spectrum of pulmonary presentations, ranging from entirely asymptomatic to fatal disease progression. For example, patients with chronic obstructive pulmonary disease (COPD) and smokers have a higher risk of severe disease and poorer outcomes. This could be because both patients with COPD and smokers have higher ACE2 receptor expression than controls, as it is believed that smoking can
upregulate ACE2 expression on epithelial cells and type 2 pneumocytes.10 Other populations that are vulnerable to poorer disease outcomes with SARS-CoV-2 include the elderly, males, and those with severe obesity, although the reasoning behind this is not well established yet.11 Common comorbidities associated with severe disease progression in COVID-19 include diabetes, hypertension, cardiac arrhythmias, and kidney failure.12 According to a cohort study investigating risk factors for severe disease in COVID-19, older age, neutrophilia, organ and coagulation dysfunction, and high D-dimer were all associated with a higher likelihood of developing acute respiratory distress syndrome (ARDS) and progressing to death.13
upregulate ACE2 expression on epithelial cells and type 2 pneumocytes.10 Other populations that are vulnerable to poorer disease outcomes with SARS-CoV-2 include the elderly, males, and those with severe obesity, although the reasoning behind this is not well established yet.11 Common comorbidities associated with severe disease progression in COVID-19 include diabetes, hypertension, cardiac arrhythmias, and kidney failure.12 According to a cohort study investigating risk factors for severe disease in COVID-19, older age, neutrophilia, organ and coagulation dysfunction, and high D-dimer were all associated with a higher likelihood of developing acute respiratory distress syndrome (ARDS) and progressing to death.13
The first cells to be infected by SARS-CoV-2 are likely to be airway cells in either the nasopharynx or trachea, which express ACE2 receptors. Although in some cases the infection does not progress any further, in other cases, the virus can spread to the lower respiratory tract, either directly or indirectly via inhalation of viral particles from the upper airway to the lower respiratory tract. In the lower respiratory tract, the ciliated cells are mostly affected. Ciliated cells in the respiratory tract contribute to transporting mucus upward along the airways. Infection of these cells can lead to loss of ciliation, and thus can disrupt the propelling of mucus upward, promoting the distribution of viral particles distally toward the alveoli. Infection of the alveolar cells usually induces type I and type III interferon (IFN) responses, which are central to an antiviral response.14 Direct viral recognition can also happen by immune cells via toll-like receptors (TLRs) such as TLR3, TLR7, TLR8, and TLR9. Interestingly, TLR7 gene defects have been associated with severe COVID-19 in young men.7
Interferon Responses
Poor type I or III IFN responses have been linked to the development of severe disease, as well as the distribution of SARS-CoV-2 to the lower respiratory tract cells. Type I IFN production specifically was found to be suppressed in patients with severe COVID-19, which seem to have a proinflammatory presentation that is driven by nuclear factor-κB (NF-κB), with high interleukin (IL)-6 and tumor necrosis factor (TNF)-α. The mechanism behind impaired production of type I IFN may be due to inborn errors of type I IFN signaling or antibodies against type I IFN, notably against IFN-α or IFN-ω. It was noted that the presence of these autoantibodies was higher in males and the elderly with severe COVID-19.15 Poor induction of IFN responses as well as the number of plasmacytoid dendritic cells, which usually secrete large amounts of type I IFNs, have been linked to aging. This could also explain why children on average present with milder cases of COVID-19, as they tend to have stronger antiviral responses against SARS-CoV-2 than adults. Thus, delayed or insufficient IFN responses could underlie the development of severe disease and subsequent lung pathology.14 On the other hand, IFN-γ, which belongs to the type II IFN family, seems to be produced in large amounts in patients with moderate to severe COVID-19. Notably, solely the combination of IFN-γ and TNF-α was able to induce PANoptosis in bone marrow–derived macrophages (BMDMs), a process characterized by a combination of pyroptosis, apoptosis, and necroptosis. This relationship could play an important role in cytokine storm seen in severe cases of COVID-19.15
Acute Respiratory Distress Syndrome
Severe COVID-19 can progress to ARDS. With time, the opacities caused by the virus are expected to resolve; however, with progression to ARDS, the opacities usually become denser and larger, and can progress to form fibrotic bands.8 The pathogenesis of COVID-19-induced ARDS is complex but likely to be attributed to the direct effects of the virus on the lung tissue and its indirect effects on the immune system.
ACE2 is abundant in bronchial epithelial cells and type I and II pneumocytes.6 It is believed that ACE2 helps protect against acute lung injury, with the binding of SARS-CoV-2 to this receptor mediating the overproduction of angiotensin II, which in turn increases pulmonary vascular permeability, leading to pulmonary edema.6
A study examining postmortem lungs of patients with COVID-19 demonstrated features of exudative and proliferative stages of diffuse alveolar damage (DAD), including capillary congestion, pneumocyte necrosis, hyaline membrane formation, type II pneumocyte hyperplasia, platelet-fibrin thrombi, and in some cases squamous metaplasia.16 Additionally, pneumocyte death was demonstrated by single-cell sequencing and immunostaining of COVID-19 lungs, which depicted a reduction in type I and II pneumocyte cells compared to controls. The mechanism by which these features form in COVID-19-induced ARDS can be explained by the immune damage of pulmonary epithelial and endothelial cells.14
Initially, the innate immune system responds to this virus through the detection of pathogen-associated molecular patterns (PAMPs) by various receptors on epithelial cells, alveolar resident macrophages, and dendritic cells. These cells respond by upregulating various inflammatory molecules, namely type 1 IFN, which can disrupt bonds between endothelial and epithelial cells, damaging cell-cell junctions. Consequently, this can increase vascular permeability and contribute to pulmonary edema, which leads to severe hypoxia and diminished removal of carbon dioxide. Under normal conditions, the lungs have mechanisms to combat pulmonary edema, as they can clear excess fluid from the alveolar space via active transport of sodium across the alveolar epithelium. However, because of the epithelial tight junction damage in acute lung injury, this process is compromised, which can lead to further edema. Furthermore, both type I and II pneumocytes are injured by the virus and the inflammation that ensues. Type I pneumocytes mainly aid in gas exchange; thus, their damage contributes to hypoxia. On the other hand, type II pneumocytes normally secrete surfactant that lines alveoli to reduce their surface tension and prevent them from collapsing,17 so their damage can invoke alveolar collapse and hypoxemia, and promote hyaline membrane formation. As a response to type II pneumocyte damage, hyperplasia of these cells occurs in the proliferative phase of ARDS. Destruction of lung epithelium and endothelium in severe cases of COVID-19 has been associated with the presence of perivascular T lymphocytes. Various studies have observed a decline in CD8+ T cells and CD4+ T-cell subsets, with the amount of CD8+ T-cell lymphopenia being inversely correlated with the degree of inflammation in COVID-19. The decline of T-cell subsets could be due to profuse T-cell activation.7
Vascular Dysfunction
Vascular dysfunction in the lungs seems to play a key role in severe COVID-19, with thrombosis being a key finding in patients. A study examining postmortem lungs of patients that died from COVID-19 demonstrated widespread vascular thrombosis with microangiopathy and occlusion of alveolar capillaries. When comparing these lungs to those of patients that died from ARDS secondary to influenza A, they found alveolar capillary microthrombi were 9 times more prevalent in patients that died of COVID-19. This study also demonstrated severe endothelial injury associated with the presence of intracellular virus, as well as significant intussusceptive angiogenesis, which could be due to a combination of the tissue hypoxia, endotheliitis, and thrombosis that was identified in COVID-19 lungs.18
The mechanism by which vascular dysfunction occurs in COVID-19 is still being investigated and is likely multifactorial. Firstly, damage to alveolar cells contributes to the activation of coagulation and inhibition of fibrinolysis, a key feature of the exudative phase in ARDS. This can result in the formation of hyaline membranes, which are fibrin-rich exudates, and can further impair gas exchange. Additionally, it can contribute to the formation of fibrin thrombi found in arteries, which is usually accompanied by high D-dimer levels and poor prognosis.14 Lung cell injury mediates the release of von Willebrand factor (vWF), which is elevated in patients with COVID-19 and can cause micro-thrombosis by binding platelets together. Thrombocytopenia is also associated with poor prognosis, likely because of the consumption of platelets in clotting. Angiopoietin-2 (Ang-2) is also released after cell injury and is known to inhibit anti-inflammatory and anticoagulatory activity, thus promoting both thrombosis and increased pulmonary vasculature permeability. Because of endothelial damage, tissue factor is released and can trigger the extrinsic coagulation pathway, leading to more formation of fibrin, as well as the intrinsic coagulation pathway via activation of factor XII.14 This micro-thrombosis is also mediated by injury to the epithelial cells, which normally have antithrombotic actions, whereas the consequences of thrombosis include disruption of gas exchange, hypoxemia, and life-threatening complications such as pulmonary embolism.
Another aspect of the immune system, the complement system, also seems to play a role in SARS-CoV-2 acute lung injury, as complement deposition in the interalveolar space promotes further pulmonary vasculature permeability, fluid leakage, and cytokine and neutrophil recruitment, continuing this cycle of inflammation. The lectin pathway, specifically, can generate complement component 5a (C5a), which can increase tissue factor expression. In patients with COVID-19, activation of peptide C5a and membrane attack complex (MAC; C5b-9) were increased in proportion to the severity of disease.7 Complement activation could also generate T cells that expressed cluster of differentiation (CD)16, which can damage endothelial cells and release inflammatory chemokines.
The recruited neutrophils release toxic substances like reactive oxygen species and neutrophil extracellular traps (NETs), the latter of which has prothrombotic actions and damages tissues and barriers. NETs can promote coagulation by recruiting more platelets and also by being directly coated in tissue factor. Research indicates that both absolute neutrophil count and a specific NET marker (myeloperoxidase [MPO]-DNA) are correlated with disease severity, decreased P/F ratio (which indicates the severity of hypoxemia), critical interventions like mechanical ventilation, and fatal outcomes.19
Macrophages
Macrophages also play a substantial role in COVID-19 pathogenesis, with both resident alveolar macrophages and recruiting macrophages being involved. Different macrophage phenotypes can be involved at different stages of the disease, and a switch from the M1 to the M2 phenotype seems to occur in the proliferative stage of the disease. In this case, the M2 macrophages seem to promote extracellular matrix (ECM) deposition and thus profibrotic actions, which could contribute to pulmonary fibrosis and macrophage activation syndrome. It has also been noted that in COVID-19, there is increased infiltration of both monocytes and macrophages, which can be dysfunctionally activated and express a multitude of inflammatory chemokines such as CCL2, CCL3, CCL20, IL-8, and TNF. Furthermore, a study investigating the cytokine storm in COVID-19 demonstrated potent dysregulation of IFN-γ, IL-6, IL-10, monocyte chemoattractant protein (MCP)-3, CXCL9, CXCL10, CXCL11, ENRAGE, poly (ADP-ribose) polymerase 1 (PARP-1), and IL-1RA. These findings indicate dysregulation of neutrophil, natural killer cells, and macrophages, which can be associated with increased severity of disease.20
Various studies have revealed that alveolar macrophages, which play a role in maintaining homeostasis, are depleted from bronchoalveolar lavage fluids in severe COVID-19, whereas proinflammatory monocyte-derived macrophages are increased.21 Additionally, alveolar macrophages were found to have decreased expression of the receptor tyrosine kinase AXL, which normally helps clear apoptotic cells to resolve inflammation.14
Cytokine Storm
The activation and recruitment of neutrophils and macrophages, and the following excessive production of cytokines, contribute to a phenomenon known as a “cytokine storm,” notably the release of proinflammatory cytokines, such as IL-6, TNF-α and IL-1. These cytokines can promote coagulation, leading to thrombi, and can directly cause lung injury and recruit further immune cells to the lungs, generating this self-perpetuating loop of thromboinflammation.19 The systemic inflammation that arises can also contribute to multiorgan failure.22 Several markers for severe disease have been identified, including C-reactive protein (CRP), which was shown to be a strong predictor of mortality in patients aged 60 years or older with COVID-19, whereas a systematic review demonstrated a correlation between high CRP levels and COVID-19 severity. Additionally, many studies have demonstrated that ferritin is a good marker for disease severity in COVID-19.7
CD24 has the ability to bind to DAMPs, preventing them from binding to pattern recognition receptors such as TLRs, thus inhibiting DAMP activation of NF-κB, a critical pathway that drives production of cytokines and chemokines. Researchers created a CD24-enriched exosome, EXO-CD24, as a therapy for cytokine storm in COVID-19. EXO-CD24 was found to effectively reduce inflammatory markers, cytokines, and chemokines, leading to inhibition of tissue injury–driven inflammation, without affecting pathogen-induced immune activation, thus potentially having a role in the future treatment of COVID-1923 (Figure 10.2).
COVID-19-Associated Infections
Apart from the detrimental effects that COVID-19 can have on the lungs, it could also be a risk factor for the development of other infections, which can further impair pulmonary function. There have been cases where patients have experienced bacterial or fungal infections during or after COVID-19 infection. Although the exact mechanism underlying this is still being researched, it is believed that the hyperinflammation involved, as well as the lymphopenia (low lymphocyte count) induced by COVID-19 may make patients susceptible to other infections. Additionally, fungal infections, such as COVID-19-associated mucormycosis (CAM) and COVID-19-associated pulmonary aspergillosis (CAPA), could be promoted because of injured epithelial cells expressing certain receptors that can interact with surface proteins on fungal pathogens, making it easier for them to adhere onto and invade host cells, causing infection.5
THE HEART
SARS-CoV-2 infection has been linked to cardiac dysfunction. Patients with preexisting cardiac aliments often present with more severe disease and respiratory symptoms, while they also may have a worse prognosis.24 Additionally, COVID-19 can cause new-onset cardiovascular disorders such as myocarditis, acute coronary syndrome (ACS), thromboembolism, arrhythmias, and heart failure, while it can also exacerbate preexisting cardiovascular conditions. SARS-CoV-2 infection has also been linked to the development of a hyperinflammatory syndrome similar to Kawasaki disease in pediatric patients, which can have cardiovascular manifestations.25 A study investigating the risks of cardiovascular outcomes in patients with COVID-19, using participants from the U.S. Department of Veterans Affairs, demonstrated that after the first 30 days postinfection, people with COVID-19 showed increased risks and 1-year burdens of incident cardiovascular diseases of various categories including cerebrovascular disorders, pericarditis, myocarditis, heart failure, thromboembolic disease, dysrhythmias, and both ischemic and nonischemic heart disease. These risks were also present regardless of age, race, sex, and cardiovascular risk factors, which indicates that these risks can present in COVID-19 patients with low risks of cardiovascular disease. Furthermore, these risks still manifested in patients that were not hospitalized during their infection.26
One study investigating cardiac manifestations in individuals with mild COVID-19 that had no previous cardiac disease or notable comorbidities found that cardiac symptoms were likely to be related to subclinical inflammatory cardiac involvement. Participants with cardiac symptoms were shown to have higher mapping values, indicating diffuse myocardial inflammation, as well as more frequent pericardial enhancement and thus pericardial inflammatory involvement, when compared to asymptomatic patients. Importantly, profound myocardial injury or structural heart disease was not a prerequisite for the presence of cardiac symptoms such as exertional dyspnea, palpitations, and chest pain.27
Myocardial Injury
Acute myocardial injury can occur because of SARS-CoV-2 infection and is associated with worse prognosis in patients. Myocardial injury in patients with COVID-19 can be associated with elevated cardiac markers or abnormal cardiac imaging studies. It can occur in a wide range of ages and is thought to occur more frequently in men. Symptoms associated with myocarditis include chest pain and dyspnea, and they most commonly present during the first 2 weeks of infection, whereas histologically, COVID-19-associated myocarditis is associated with monocyte and lymphocyte infiltration in the myocardial interstitium.28
The exact mechanism behind this is poorly understood, as there is conflicting evidence on whether SARS-CoV-2 directly infects myocardial cells or whether the myocarditis is secondary to systemic inflammation and hypoxia.
There have been reported cases of viral myocarditis where SARS-CoV-2 has infected cardiomyocytes via the ACE2 receptor. A study investigating the cardiac tropism of SARS-CoV-2 found that human pluripotent stem cell–derived cardiomyocytes that expressed ACE2 can be directly infected by SARS-CoV-2, and as a result, these infected cells show diminished contractility and electrophysiologic properties, as well as widespread cell death. Additionally, cardiac stromal cells can be infected by the virus directly and can also be converted into hyperinflammatory phenotypes, promoting myocardial damage.29 Autopsy samples from patients infected with SARS-CoV found viral RNA in heart samples, marked macrophage infiltration, and myocardial injury, demonstrating that SARS-CoV could directly infect the myocardium. Because SARS-CoV and SARS-CoV-2 share similarities in cell entry via the ACE2 receptor, this may suggest that SARS-CoV-2 could infect the heart directly in an analogous manner.25
However, there is also evidence that myocardial injury may be more commonly due to systemic inflammation instead. A study investigating patients with myocardial injury showed that they did not have the usual signs of myocarditis, such as segmental wall motion abnormalities or reduced left ventricular ejection fraction, which could imply that the myocardial injury was due to systemic causes. On the other hand, there are other cases of patients with COVID-19 demonstrating typical features of myocarditis.25
Arrhythmias
There have been cases of arrhythmias occurring in patients with COVID-19, with atrial fibrillation being the most common type, which is also associated with poor prognosis.30
Arrhythmias in COVID-19 can occur because of various mechanisms, including electrolyte disturbances, systemic inflammation, and the use of drugs with potential cardiotoxic side effects.
Firstly, the binding of SARS-CoV-2 to the ACE2 receptor enhances the activity of RAAS, leading to more production of aldosterone, which as a result can activate epithelial sodium channels (ENaC), leading to potassium loss.31 This resultant hypokalemia, which is a common clinical manifestation in COVID-19, can lead to the development of cardiac arrhythmias, predominantly via reducing the cardiac repolarization reserve and by causing an accumulation in intracellular Ca+.32
Independent of the activation of RAAS, electrolyte disturbances because of systemic illness can also promote arrhythmias, of which patients with preexisting cardiovascular problems are particularly vulnerable to.
Additionally, hypoxia, decreased coronary perfusion, direct myocardial injury, and systemic causes like sepsis are all factors that can trigger the development of cardiac arrhythmias, such as atrial and ventricular tachycardia and fibrillation. Hypoxia, which in COVID-19 is often secondary to respiratory failure or ARDS, can contribute to arrhythmias, as hypoxia can change the function
of L-type calcium channels, thus affecting the plateau phase of the action potential. The anaerobic metabolism that can occur as a result of the hypoxia reduces intracellular pH and affects the action potential duration. This can cause aberrant remodeling in the cardiac conduction tissue because of its effects on the gap junction proteins connexin 40 and connexin 43. A combination of these factors can lead to atrial and ventricular arrhythmias.
of L-type calcium channels, thus affecting the plateau phase of the action potential. The anaerobic metabolism that can occur as a result of the hypoxia reduces intracellular pH and affects the action potential duration. This can cause aberrant remodeling in the cardiac conduction tissue because of its effects on the gap junction proteins connexin 40 and connexin 43. A combination of these factors can lead to atrial and ventricular arrhythmias.
Myocardial damage has also been linked to the development of arrhythmias, as myocarditis can cause remodeling of the cardiac conduction tissue, microvascular ischemia, and can affect gap junctions and ion channels. The fibrosis that occurs after myocardial damage could also contribute to reentrant arrhythmias. The inflammatory cytokines that are released, such as IL-6 and TNF-α, can directly affect cardiac potassium and calcium channels, and thus affect the action potential, although this is usually in patients with genetic predispositions.30 A retrospective analysis of patients that were hospitalized with COVID-19 revealed that new-onset atrial fibrillation or atrial flutter was associated with inflammatory markers such as IL-6. Similarly, another study revealed that high levels of IL-6 and IL-10 were predictive of the risk of atrial and ventricular arrhythmias in patients with COVID-19.33
Furthermore, various drugs that had been used as a treatment for COVID-19, especially at the start of the pandemic, increased the risk of arrhythmias. For example, a combination of hydroxychloroquine and azithromycin was used at the start of the pandemic because of their antiviral effects; however, these medications increased the risk of arrhythmias by prolonging the QT interval. Additionally, remdesivir, which is still used as an antiviral for COVID-19, can lead to bradyarrhythmia.30
During the convalescent period of COVID-19 infection, dysautonomia has also been documented and is thought to be due to postinfectious autoimmunity30 (Figure 10.3).
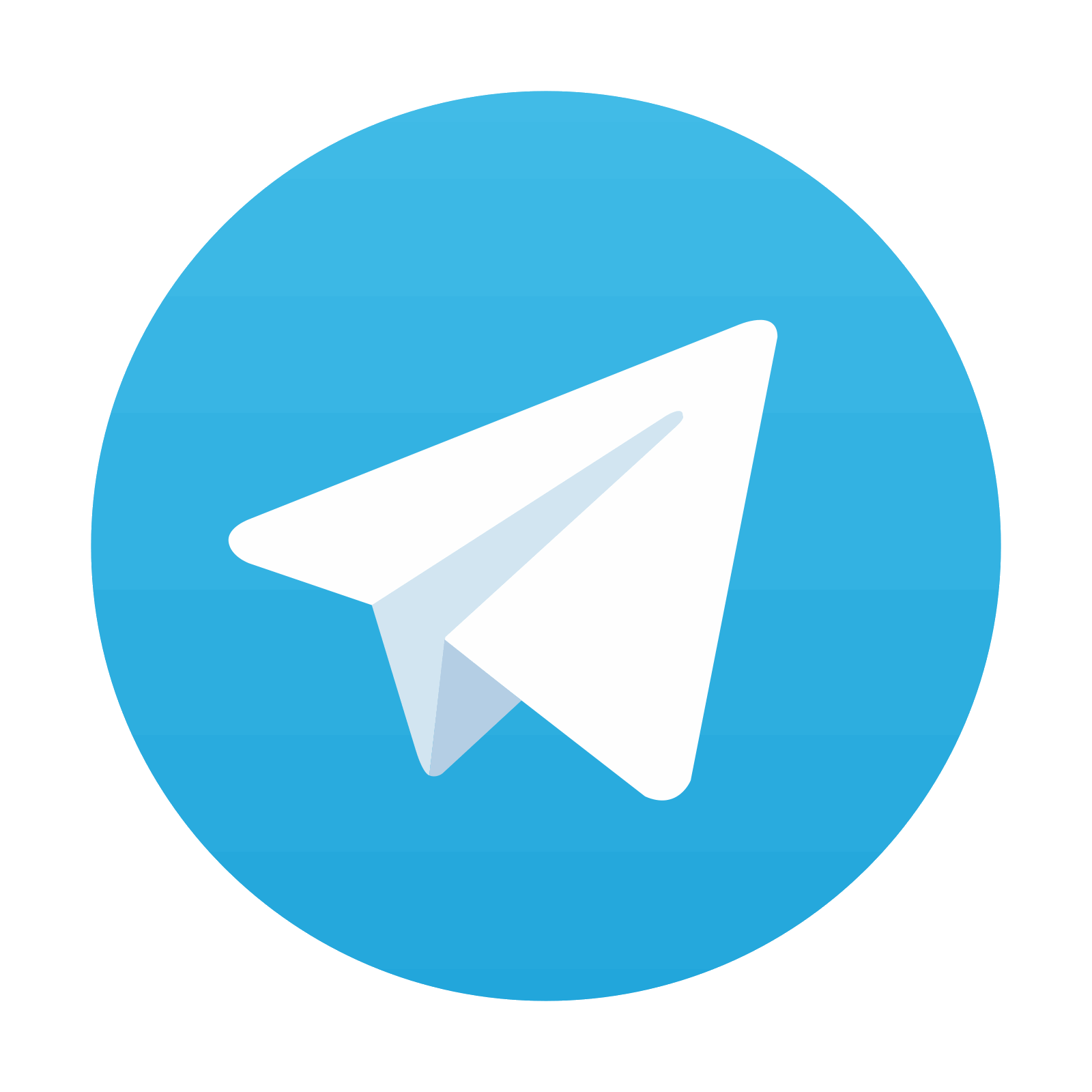
Stay updated, free articles. Join our Telegram channel
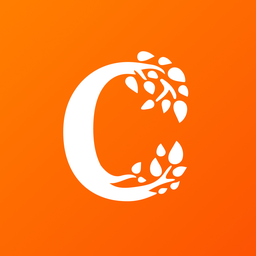
Full access? Get Clinical Tree
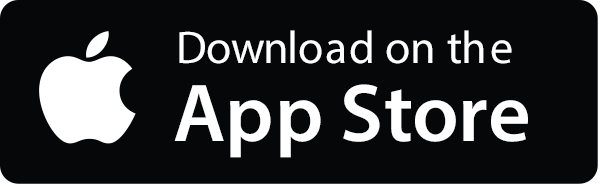
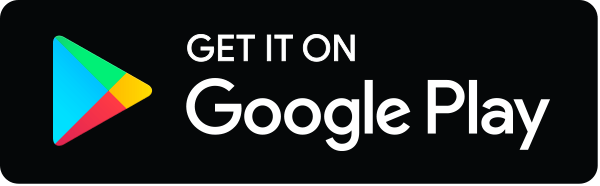