Nonfermentative Gram-Negative Bacilli
Valentina Stosor
Alan R. Hauser
John P. Flaherty
Nonfermentative gram-negative bacilli are a diverse array of microorganisms that have evolved in aquatic environments, have minimal growth requirements, and differ substantially in virulence. Included in this category are Pseudomonas aeruginosa, other Pseudomonas species, and genera such as Stenotrophomonas, Acinetobacter, Burkholderia, Flavobacterium, and Achromobacter. During the last half-century, nonfermentative gram-negative bacilli have become significant healthcare-associated pathogens because of the many reservoirs they inhabit in hospitals and the resistance of these microorganisms to commonly used antibiotics. Furthermore, the virulence of P. aeruginosa and its frequency as a healthcare-associated pathogen have been important factors impelling increased use of antipseudomonal β-lactam antibiotics and quinolones as presumptive therapy where P. aeruginosa is a potential pathogen.
MICROORGANISM CHARACTERISTICS
Pathogenicity
Like other bacterial pathogens, nonfermentative gram-negative bacilli must successfully navigate through a series of critical steps to cause disease. After entering the host, bacteria attach to host cells, breach host barriers, access nutrients to proliferate, evade local host defenses, and in some cases spread to regional or distant sites (1). To accomplish these tasks, bacteria produce a number of cell-associated and secreted virulence determinants in a tightly regulated manner. Pathogenesis has been studied most extensively in P. aeruginosa, and this bacterium will be used as a model in our discussion of pathogenesis. However, it should be noted that each of the nonfermentative gram-negative bacilli has evolved its own unique set of pathogenicity factors and accomplishes the difficult process of causing infection by a different means.
Regardless of the type of infection, bacterial attachment to host epithelial cells is typically a prerequisite to the pathogenesis of bacterial disease. Attachment of P. aeruginosa (formerly P. pyocyanea) is accomplished by a number of surface structures including type IV pili (or fimbriae), flagella, lipopolysaccharide, and lectins. Of these, type IV pili have received the most attention as adhesins (2). These polar filaments are composed of repeated monomers of the protein pilin and bind to the GalNacβ(1, 2, 3 and 4)Gal moiety of asialylated glycolipids found on epithelial cells (3). Disruption of pilus biosynthetic genes results in decreased virulence in animal models of acute pneumonia (4,5).
Despite producing a number of adhesins, P. aeruginosa attaches to the apical surface of intact epithelial cells quite poorly (6). P. aeruginosa is thus blocked at this very early step in the pathogenic process, an observation that likely explains why healthy individuals are rarely infected with this ubiquitous bacterium. However, in a host in which skin and mucosal surfaces are disrupted by endotracheal intubation and mechanical ventilation, burn wounds, corneal abrasion, indwelling catheters, or cytotoxic chemotherapy, P. aeruginosa has all the virulence machinery necessary to proceed through the remaining steps of pathogenesis and to cause severe infection. Injury of epithelium leads to the loss of polarized distribution of cellular ligands for P. aeruginosa. In particular, heparin sulfate proteoglycans normally found only on the basal-lateral surface of epithelial cells are redistributed to the apical surface following tissue damage (7). These proteoglycans are avidly bound by P. aeruginosa adhesins and allow this bacterium to efficiently bind to the apical surface of damaged epithelium.
Once P. aeruginosa bacteria successfully bind to a surface (living or inert), they are capable of forming biofilms under appropriate conditions. Biofilms are communities of microbes embedded in an organic polymer matrix at an air-solid or liquid-solid interface. The extracellular matrix of biofilms formed by P. aeruginosa consists of exopolysaccharides, proteins, and extracellular DNA. In particular, three exopolysaccharides play an important role in biofilm formation and maintenance: Psl, Pel, and alginate (8). The extracellular matrix confers partial resistance to the host immune response by inhibiting antibody coating, phagocytosis, and intracellular killing by leukocytes (9, 10, 11 and 12). Thus, even in individuals with normal cellular and humoral immune function, biofilm-related infections are rarely resolved by the host defense mechanisms (9). Unfortunately, biofilms are also quite resistant to eradication by antimicrobial agents. Although the reasons for this are unclear, three mechanisms have been proposed. First, antimicrobial agents may diffuse slowly through the extracellular matrix of a biofilm, resulting in subinhibitory concentrations within the biofilm interior (13,14). Second, bacteria in certain regions of the biofilm may assume a metabolically or otherwise inactive state
that allows persistence in the presence of antibiotics (15). Third, zones of biofilms may accumulate waste products and have low oxygen tensions, conditions that antagonize the activity of some antibiotics (16). More recently, it has been shown that mutations and horizontal transfer of genetic material occur more frequently during a biofilm mode of growth (17,18). All these processes could contribute to the ability of P. aeruginosa biofilms to develop antimicrobial resistance and to persist despite antibiotic therapy. P. aeruginosa biofilms play important roles in the pathogenesis of central venous catheter-related infection, urinary catheter cystitis, contact lens-associated corneal infection, lung infection in cystic fibrosis, and ventilatorassociated pneumonia (VAP) (9). In these infections, antibiotic therapy typically eliminates the symptoms caused by the planktonic cells released from the biofilm but fails to kill the biofilm itself. As a result, biofilm infections typically show recurring symptoms until the sessile population of bacteria along with the infected catheter or prostheses is removed.
that allows persistence in the presence of antibiotics (15). Third, zones of biofilms may accumulate waste products and have low oxygen tensions, conditions that antagonize the activity of some antibiotics (16). More recently, it has been shown that mutations and horizontal transfer of genetic material occur more frequently during a biofilm mode of growth (17,18). All these processes could contribute to the ability of P. aeruginosa biofilms to develop antimicrobial resistance and to persist despite antibiotic therapy. P. aeruginosa biofilms play important roles in the pathogenesis of central venous catheter-related infection, urinary catheter cystitis, contact lens-associated corneal infection, lung infection in cystic fibrosis, and ventilatorassociated pneumonia (VAP) (9). In these infections, antibiotic therapy typically eliminates the symptoms caused by the planktonic cells released from the biofilm but fails to kill the biofilm itself. As a result, biofilm infections typically show recurring symptoms until the sessile population of bacteria along with the infected catheter or prostheses is removed.
Once a focus of infection is established, P. aeruginosa employs its broad spectrum of metabolic pathways to utilize nutrients available within the host. One such essential nutrient is iron, which is tightly sequestered by a number of host factors. P. aeruginosa secretes two siderophores named pyoverdin and pyochelin to access these cellular stores (19). Both are pigmented diffusable molecules that chelate iron in the environment and then transport it into the bacterium via specific outer membrane receptors. The yellow-green fluorescence of pyoverdine is sometimes apparent in wounds and dressings, allowing a presumptive diagnosis of P. aeruginosa infection to be made (20). Interestingly, P. aeruginosa has the ability to hijack and utilize siderophores secreted by other microrganisms (21).
After overcoming the initial obstacles to infection, P. aeruginosa employs a number of secretion systems to export toxic factors into the host environment. These factors function to impair host immune components or disrupt host barriers to facilitate dissemination. Here we will review each of these secretion systems in turn.
Type I secretion is a relatively simple three-component mechanism used by gram-negative bacteria to export a dedicated factor from within the bacterium to the extracellular environment. P. aeruginosa uses such a system to export alkaline protease, an enzyme that cleaves components of the complement cascade and the extracellular matrix (22,23). Animal models of keratitis and burn infections have demonstrated that alkaline protease plays an important role in the pathogenesis of these infections (24).
A number of P. aeruginosa virulence factors are exported by a type II secretion system, which similarly functions to move bacterial proteins across the inner and outer bacterial membranes. P. aeruginosa factors secreted by this system include exotoxin A, phospholipase C, and elastase. Exotoxin A is a secreted adenosine ribosyltransferase that inhibits protein synthesis in eukaryotic cells by modifying the structure of elongation factor-2 (25). Exotoxin A is produced by most clinical isolates of P. aeruginosa and has potent local and systemic effects. These include necrosis of soft tissues into which sublethal doses are injected (26) and shock and hepatocellular necrosis following systemic administration (27). Although experimental models of local infection have not consistently shown enhanced virulence of P. aeruginosa isolates that produce exotoxin A (28,29), studies of bacteremic infection in humans suggest an important role (30,31).
Elastase, a metalloprotease that accounts for the majority of the proteolytic activity of P. aeruginosa, cleaves elastin as well as a number of other host factors. One such factor is syndecan-1, a heparin sulfate proteoglycan found on the surface of respiratory mucosal cells (32). It has been postulated that P. aeruginosa utilizes the shed portion of cleaved syndecan-1 to protect itself from host defenses (32). Animal models support a pathogenic role for elastase, especially in the lung (33).
P. aeruginosa produces two phospholipase C enzymes, sometimes referred to as Plc-HR and Plc-N. These enzymes can degrade surfactant in the lung and cause tissue destruction (34). Mutants with disruptions in the genes encoding these phospholipases were defective in virulence in a mouse burn model (35).
All P. aeruginosa strains also encode a type III secretion system, although this system appears to not function in some isolates. The type III secretion system uses a complex secretion/translocation mechanism to move toxins (called effector proteins) directly from the bacterium into host cells. Four effector proteins have been identified in P. aeruginosa: ExoS, ExoT, ExoU, and ExoY. ExoS and ExoT are related proteins that have ADP-ribosyltransferase and GTPase-activating protein activities. These activities are directed against a number of host proteins and are thought to inhibit phagocytosis, disrupt epithelial barriers, and cause apoptosis (36,37). ExoU is a potent phospholipase that causes rapid necrotic cell death of a number of cell types, including neutrophils during acute pneumonia (38, 39 and 40). ExoY is an adenylate cyclase that elevates intracellular cyclic adenosine monophosphate levels (41). ExoU and ExoS have been shown to be important virulence factors in several animal models (40,42). Type III secretion itself has been associated with worse clinical outcomes in patients with VAP and bloodstream infection (43,44).
P. aeruginosa does not harbor a type IV secretion system and the relationships between this bacterium’s type V secretion systems and virulence have not been examined in detail. Recently, however, examples of a newly described type VI secretion system have been found in P. aeruginosa (45,46). One such system, designated H1-T6SS, has been shown to secrete three substrates: Tse1, Tse2, and Tse3 (47). Work is ongoing to determine whether this secretion system is directed against eukaryotic microorganisms such as humans or competing bacteria, or both (47).
P. aeruginosa produces a number of additional factors that have been implicated in disease pathogenesis, including rhamnolipids, pyocyanin, and leukocidin. The reader is referred to several recent reviews for more detailed information on these factors and P. aeruginosa pathogenesis in general (48,49).
As would be expected, complex, integrated, and overlapping regulatory networks are required to ensure that many P. aeruginosa virulence determinants are activated at the appropriate time and place during infection. One such regulatory system is cyclic-diguanylate. This second messenger molecule is synthesized from guanosine triphosphate by diguanylate cyclases and degraded by
phosphodiesterases. Interestingly, more than 30 of these enzymes are believed to be encoded by the P. aeruginosa genome, suggesting complex regulation of this second messenger (50). Cyclic-diguanylate levels within the bacterium, to a large extent, control biofilm formation by regulating matrix production and adhesion (51,52).
phosphodiesterases. Interestingly, more than 30 of these enzymes are believed to be encoded by the P. aeruginosa genome, suggesting complex regulation of this second messenger (50). Cyclic-diguanylate levels within the bacterium, to a large extent, control biofilm formation by regulating matrix production and adhesion (51,52).
A more global regulatory system employed by P. aeruginosa is quorum sensing. Quorum sensing allows bacteria to detect the density of their own species and alter their gene expression patterns to take advantage of this density. Small diffusible signal molecules called autoinducers are secreted by P. aeruginosa. At a specific cell density, the concentration of these molecules becomes sufficient to activate transcriptional regulator proteins and induce gene transcription. In vitro, quorum-sensing systems modulate expression of 6% to 10% of the genes in the P. aeruginosa genome (53,54). P. aeruginosa produces several autoinducers, but two small acyl-homoserine lactone molecules referred to as PAI-1 and PAI-2 have been most extensively studied (55). PAI-1 activates the LasR protein, which enhances the transcription of extracellular virulence factors including alkaline protease, exotoxin A, and elastase. The formation of a normal, dense biofilm is also dependent on the synthesis of PAI-1 (56,57). The autoinducer molecule PAI-2 binds RhlR and initiates transcription of several different genes involved in a variety of adaptations including biofilm formation. A further indication of the complexity of regulation is that the Las system itself regulates the Rhl system. P. aeruginosa strains with inactivated quorum-sensing systems demonstrate significantly diminished virulence in animal models of infection (58).
Another global regulatory system used by P. aeruginosa consists of RetS and LadS. These two proteins are reciprocally activated hybrid sensor kinases/response regulators that form a molecular switch priming P. aeruginosa for either an acute (RetS) or a chronic (LadS) infection lifestyle. They activate downstream pathways that posttranslationally regulate a number of virulence determinants, including type III secretion, piliation, and a biofilm mode of growth (59, 60, 61 and 62). Thus, RetS and LadS may sense environmental cues consistent with acute infection (e.g., VAP) or chronic infection (cystic fibrosis) and alter gene expression accordingly.
During pathogenesis, P. aeruginosa pathogenicity factors interact intimately with the host’s immune system to cause pathology (63,64). Key components of immunity to invasive infection are TLRs (65), the inflammasome (66,67), polymorphonuclear leukocytes (68,69), and antibodies against the lipopolysaccharide cell wall (70,71). A role for cellular immunity (in particular a Th17 response) is also suggested by reports of invasive pseudomonal infection in patients with cellular immune impairment (72,73) and is supported by experimental findings involving T cells (74,75).
By most measures, Stenotrophomonas maltophilia (formerly Pseudomonas maltophilia and then Xanthomonas maltophilia) is not particularly virulent (reviewed in Ref. 76). In the burned mouse model, inocula of 3 × 107 colonyforming units (CFU)/mL of S. maltophilia failed to establish lethal infection (70). In contrast, only 2 × 102 CFU/mL of P. aeruginosa caused fatal infection in all the animals studied. Nevertheless, S. maltophilia can cause serious infection in compromised hosts. Genomic comparison of a clinical S. maltophilia isolate to an environmental isolate demonstrated that the clinical isolate encoded a unique filamentous hemagglutinin, a protein that functions as an adhesin in other bacteria, and a type IV pilus (77). S. maltophilia also produces or carries genes encoding a number of putative virulence determinants, including protease, elastase, DNase, lipase, fibrinolysin, and zonula occludens toxin (78, 79 and 80). The latter is similar to an enterotoxin produced by Vibrio cholerae. Consistent with the production of these factors, S. maltophilia was cytotoxic toward a number of mammalian cell lines (81) and lethal in a Caenorhabditis elegans infection model (82). Particularly, significant protease and elastase production was identified in a clinical isolate of S. maltophilia from a leukemic patient with bacteremia and ecthyma gangrenosum, mimicking both the clinical and virulence properties of P. aeruginosa (83).
The Burkholderia cepacia complex group of bacteria was originally described in 1950 as a cause of soft rot in onions (Latin: coepa) and previously named eugonic oxidizer group 1, Pseudomonas kingii, Pseudomonas multivorans, Pseudomonas P. alcaligenes IVc, and Pseudomonas cepacia. More recently, they were given the name B. cepacia, but as these microorganisms were further characterized, it became clear that B. cepacia actually comprised a group of several related but distinct bacteria that have now been given species designations. Currently this group of bacteria is referred to as the B. cepacia complex and consists of more than 16 species (84, 85, 86 and 87) (reviewed in 88). These bacteria are extremely versatile and capable of utilizing a wide variety of nutrients for growth. They thrive in natural water sources and can proliferate in tap or distilled water, presumably by utilizing trace elements and low concentrations of organic materials (89, 90, 91, 92 and 93). Like S. maltophilia bacteria, B. cepacia complex strains can cause significant disease in the compromised patient and produce a number of important virulence factors. The cable pilus, an adhesin, is used to tether bacteria to cytokeratin 13 on the surface of host cells (94). B. cepacia complex bacteria can enter and survive intracellularly in cultured macrophages and pulmonary epithelial cells, which may provide a protected niche, allowing persistent infection (95). Flagella are necessary for the invasion process, and mutants lacking functional flagella were defective in invasion in vitro and in lethality in a mouse model of chronic pulmonary infection (96,97). B. cepacia complex bacteria also have a quorumsensing system (CepIR), which is necessary for biofilm formation, secretion of putative toxins, and full virulence in a rodent model (98,99). Interestingly, the B. cepacia complex quorum-sensing system also responds to autoinducers from P. aeruginosa (100), demonstrating interspecies communication. Some strains within the B. cepacia complex contain a pathogenicity island named the cenocepacia island (101). This island encodes a second quorum-sensing system (CciIR), metabolic pathways, and several transcriptional regulators. Mutations in specific portions of this island caused defects in bacterial persistence and inflammation in a rat chronic infection model (101).
Acinetobacter, formerly classified as Mima, Herellea, Moraxella, Neisseria, Bacterium, Alcaligenes, Achromobacter, and Pseudomonas, is widely distributed in nature and is part of the normal flora of many animal species and humans
(102,103, reviewed in Ref. 104). By DNA hybridization, there may be as many as 17 species (105), of which Acinetobacter baumannii is the most clinically relevant. A. baumannii is an uncommon pathogen (106,107) that usually infects immunocompromised or debilitated hosts and sometimes causes outbreaks in intensive care units (ICUs) (108, 109 and 110). A number of A. baumannii factors likely to be important in pathogenesis have been identified including siderophores for iron acquisition (111,112), piluslike structures that facilitate adherence to host cells and biofilm formation (113,114), and multiple quorum-sensing systems (115). The sequencing of the A. baumannii genome identified a substantial number of genomic islands (116,117), six of which were shown to enhance virulence in C. elegans and Dictyostelium discoideum models of infection (117). The virulence-associated islands contained genes predicted to encode transcription factors, multidrug efflux transport systems, and a urease.
(102,103, reviewed in Ref. 104). By DNA hybridization, there may be as many as 17 species (105), of which Acinetobacter baumannii is the most clinically relevant. A. baumannii is an uncommon pathogen (106,107) that usually infects immunocompromised or debilitated hosts and sometimes causes outbreaks in intensive care units (ICUs) (108, 109 and 110). A number of A. baumannii factors likely to be important in pathogenesis have been identified including siderophores for iron acquisition (111,112), piluslike structures that facilitate adherence to host cells and biofilm formation (113,114), and multiple quorum-sensing systems (115). The sequencing of the A. baumannii genome identified a substantial number of genomic islands (116,117), six of which were shown to enhance virulence in C. elegans and Dictyostelium discoideum models of infection (117). The virulence-associated islands contained genes predicted to encode transcription factors, multidrug efflux transport systems, and a urease.
The pathogenesis or virulence properties of other nonfermenters have not been fully elucidated. Sphingomonas paucimobilis appears to have limited inherent virulence, but it does have endotoxin activity and produces alkaline and acid phosphatases and several esterases (118,119). A report of Shewanella putrefaciens causing refractory ulcerative cellulitis and septic shock suggested possible exotoxin production (120).
Resistance to Antimicrobial Agents
P. aeruginosa and other nonfermentative gram-negative bacilli are resistant to many common antibiotics, including first- and second-generation cephalosporins. Based on recent surveillance studies, piperacillin/tazobactam, carbapenems, piperacillin, ceftazidime, cefepime, and the polymyxins remain the most active antipseudomonal agents (104,121, 122, 123, 124, 125, 126, 127 and 128). Increased resistance across many classes of antimicrobial agents and multiclass drug resistance is a concerning trend exhibited by all the clinically important nonfermentative gram-negative bacteria (121, 122 and 123,129). Mechanisms of resistance to each class of antibiotics are diverse, and more than one mechanism may contribute to resistance to some antibiotics (130). Antimicrobial resistance exhibited by the clinically important nonfermentative gram-negative pathogens is discussed below. Other nonfermenters that are less commonly encountered have varying antimicrobial susceptibility patterns (127,131, 132, 133 and 134). Treatment of infections caused by these pathogens is typically guided by susceptibility testing of individual isolates.
For P. aeruginosa, inherent mechanisms such as outer cell membrane permeability factors and active drug efflux systems result in reduced susceptibility to many antimicrobial agents (135,136). Efflux pumps play a role in reduced susceptibility to sulfonamides, tetracycline, macrolides, fluoroquinolones, penicillins, cephalosporins, meropenem, and even the aminoglycosides (137). All P. aeruginosa inherently produce the AmpC β-lactamase that hydrolyzes penicillins and cephalosporins (136,138,139). In addition, acquired β-lactamases are responsible for penicillin, and first- and second-generation cephalosporin resistance (136,139). Production of a number of extended-spectrum β-lactamases is reported with increasing frequency, resulting in resistance to advanced-generation cephalosporins, monobactams, and extended-spectrum penicillins, depending on the enzyme(s) present (136,139). Changes in penicillin-binding proteins are a relatively uncommon mechanism of β-lactam resistance in P. aeruginosa (130,135). Historically, reduced activity of carbapenems, when present, was attributed to reduced accumulation of the drug via downregulation of carbapenem-specific porin production (137); however, carbapenemase production has been reported with increasing frequency (124,140, 141 and 142). Aminoglycosides are inactivated by enzymatic modification via the acquisition of plasmids carrying any of a number of such enzymes. The prevalence of fluoroquinolone resistance among P. aeruginosa is remarkably high (121, 122 and 123,128); mutations in the topoisomerase genes, gyrA and parC, are the genetic basis for such resistance (143, 144, 145 and 146) in addition to resistance caused by active efflux and membrane impermeability.
S. maltophilia demonstrates high-level resistance to many antimicrobial agent classes including the β-lactams, tetracyclines, and aminoglycosides (127,147). As a rule, the carbapenems are hydrolyzed by a chromosomally encoded zinc-dependent β-lactamase possessed by most strains, thus rendering these agents ineffective for the treatment of S. maltophilia infections. The most reliable agents include trimethoprim/sulfamethoxazole, ticarcillin/clavulanate, and the newer generation fluoroquinolones such as levofloxacin, moxifloxacin, or gatifloxacin (125,126,129,147, 148, 149 and 150). In addition, tigecycline demonstrates in vitro activity and polymyxin
B has variable activity against Stenotrophomonas (151,152). B. cepacia complex strains are resistant to most antibiotics commonly used for treatment of gram-negative bacterial infections, including the extended-spectrum penicillins and aminoglycosides (153). The most active antimicrobial agents are trimethoprim/sulfamethoxazole, the carbapenems, ceftazidime, and the fluoroquinolones (127,154). Burkholderia demonstrates in vitro susceptibility to tigecycline (151). Most strains are resistant to polymyxin B (127,152). Typically, serious infections are treated with combinations of antimicrobial agents.
Acinetobacter varies substantially in its susceptibility to specific antibiotics, and resistance has steadily increased over the last 3 decades (104,123,124,128,140,155). Multiple drug resistance has emerged (104), and outbreaks caused by Acinetobacter strains that are resistant to imipenem, ceftazidime, amikacin, and other routinely tested antibiotics are increasingly reported (156,157, 158, 159 and 160). The most appropriate therapy in such circumstances is unclear and should be guided by the results of antimicrobial susceptibility testing. Sulbactam exhibits antimicrobial activity against Acinetobacter, and thus, ampicillin-sulbactam may be considered for therapy (105,157,161,162). Tigecycline possesses in vitro activity against Acinetobacter (163), but data are limited regarding clinical efficacy and cases of tigecycline resistance have been reported (164,165). The polymyxins, such as polymyxin B, colistin, and similar investigational peptides, demonstrate in vitro activity against Acinetobacter and Pseudomonas and offer a potential therapy for infections caused by strains that are resistant to all other agents (157,166). Finally, there is limited data that support the use of combination therapy with agents such as colistin plus rifampin or colistin plus a carbapenem (104,167).
Antimicrobial resistance exhibited by the nonfermentative gram-negative bacilli creates an epidemiologic niche for these pathogens that facilitates colonization and
superinfection in antibiotic-treated patients. For example, multiple studies demonstrate that patients who receive antibiotics lacking anti-Pseudomonas activity are at risk for intestinal colonization (168, 169, 170 and 171) and bloodstream infections (172) caused by this bacterium. Similarly, administration of broad-spectrum antibiotics lacking activity against other nonfermentative gram-negative bacilli including Acinetobacter and S. maltophilia predisposes toward colonization and infection with these pathogens (173, 174, 175, 176 and 177).
superinfection in antibiotic-treated patients. For example, multiple studies demonstrate that patients who receive antibiotics lacking anti-Pseudomonas activity are at risk for intestinal colonization (168, 169, 170 and 171) and bloodstream infections (172) caused by this bacterium. Similarly, administration of broad-spectrum antibiotics lacking activity against other nonfermentative gram-negative bacilli including Acinetobacter and S. maltophilia predisposes toward colonization and infection with these pathogens (173, 174, 175, 176 and 177).
Resistance to Biocides
Resistance to biocides is a feature of some nonfermentative gram-negative bacilli, most notably P. aeruginosa and B. cepacia. This problem was initially recognized in the 1950s when Pseudomonas contamination of dilute aqueous benzalkonium chloride was reported at several hospitals (178,179). At these hospitals, the use of contaminated aqueous benzalkonium chloride to disinfect intravascular catheters and needles caused outbreaks of Pseudomonas bacteremia. The continued use of aqueous benzalkonium chloride alone or with other agents for antiseptic preparation of the skin or urethral meatus before catheter insertion resulted in further cases of bloodstream or urinary tract infection (180, 181, 182, 183, 184 and 185). Most outbreaks involved aqueous benzalkonium chloride diluted in hospitals to an in-use concentration of 1:1,000; however, in several instances, B. cepacia was an intrinsic contaminant of commercially prepared swabs containing a 1:500 concentration of aqueous benzalkonium chloride (183,186).
Other antiseptic preparations such as chlorhexidine (187, 188, 189 and 190), chlorhexidine and cetrimide (191,192), cetrimide (193), hexachlorophene (194), and green soap (195) have become contaminated with Pseudomonas, Burkholderia, Ralstonia, or Stenotrophomonas. While the presence of organic materials enhances survival of bacteria in these antiseptics (178,193,196), Pseudomonas and related nonfermenters may proliferate substantially in antiseptics in the absence of such contaminants (193,196). These bacteria may also contaminate phenolic disinfectants (196, 197 and 198). Even commercial preparations of poloxamer-iodine and povidone-iodine have become contaminated with P. aeruginosa (199) and B. cepacia (200, 201 and 202); in such reports, products from different manufacturers were implicated, and concentrations of free iodine, when tested, were sometimes in the same range as uncontaminated products (201,202).
The susceptibility of P. aeruginosa and B. cepacia to commonly used antiseptics and disinfectants varies. Some strains are resistant to in-use dilutions of benzalkonium chloride, chlorhexidine, cetrimide, phenolic disinfectant, iodophor disinfectant, or quaternary ammonium disinfectant (203, 204, 205, 206, 207 and 208). There are limited data regarding biocide resistance in other nonfermentative gram-negative bacteria. Two such studies examining in vitro susceptibility of A. baumannii, including multidrug resistant isolates, to biocides demonstrated no resistance to commonly used antiseptics such as chlorhexidine (209,210). In contrast, Acinetobacter isolates possessing class 1 integrons were associated with both biofilm formation and resistance to biocides including benzalkonium chloride and chlorhexidine (211).
It is evident that gram-negative bacteria may possess multiple intrinsic and acquired mechanisms for antiseptic and disinfectant resistance (212,213). P. aeruginosa and B. cepacia within biofilms are more resistant to biocides of all types, including antibiotics, than their free-living counterparts (9,214, 215, 216, 217, 218 and 219). Studies of iodophor contamination by P. aeruginosa and B. cepacia demonstrate the potential protective role of biofilm matrix (220, 221 and 222). The ways in which bacteria within a biofilm evade the actions of antimicrobials and disinfectants remain under investigation but appear identical to the mechanisms by which biofilms inhibit antibiotic activity. First, biofilms act as a permeability barrier to biocides (9,215,223). For example, in vitro resistance to povidone-iodine is mediated by the protective layering of Pseudomonas bacterial cells within a biofilm (224). In vitro, higher concentrations of biocides can overcome this type of resistance (225). Second, bacteria within a biofilm have slower growth rates that result in reduced efficacy of antimicrobials such as β-lactams.
Additional inherent and acquired mechanisms may allow evasion of biocide effects. The relative impermeability of the bacterial outer membrane may result in low-level resistance to hydrophobic compounds such as triclosan, and acquired alterations in bacterial outer membrane proteins may confer resistance to higher concentrations of such compounds (213,216,223,226). The expression of multidrug efflux pumps is another proposed mechanism of biocide resistance (137,213,223,226, 227 and 228). Resistance of Pseudomonas species to other compounds such as phenols may be mediated by catabolic enzymes (229, 230 and 231). Plasmid-mediated resistance to silver has occurred in Pseudomonas stutzeri (232).
Replication and Survival
Species of Pseudomonas and B. cepacia complex replicate in a wide range of moist environments because of their ability to obtain carbon and nitrogen from diverse substrates. Aliphatic amides or amino acids provide the source of both carbon and nitrogen. Alternatively, carbon may be derived from organic acids or esters of organic acids, whereas nitrogen is extracted from ammonium or nitrate. The range of substrates includes both antibiotics and germicides. For example, penicillin has served as a carbon source for B. cepacia (233) and Pseudomonas fluorescens (234); chlorinated phenols were utilized by an isolate-designated Pseudomonas species B 13 (235); and chlorhexidine and cetrimide were utilized by B. cepacia (191). In addition, ammonium acetate-buffered benzalkonium chloride has supported the growth of P. aeruginosa (236).
The minimal nutrient requirements of P. aeruginosa and B. cepacia complex permit their growth in tap water and distilled or deionized water up to concentrations of 105 to 107 CFU/mL (185,237, 238 and 239). Presumably, organic compounds absorbed in water from plumbing and storage systems are utilized as substrates. Naturally occurring pseudomonads in these minimal medium environments are more resistant to chemical inactivation by agents such as chlorine or iodine than are bacteria grown in enriched media (191,201,237,238).
Pseudomonads are capable of prolonged survival in moist or dry environments. In laboratory studies, P. aeruginosa can survive in water for more than 300 days, on dry filter paper disks for up to 150 days, on hardened plaster of Paris bandages for at least 20 days, on plastics used in the hospital environment for up to 2 days, and in dried sputum for at least 5 days (240, 241, 242 and 243). In the hospital environment,
P. aeruginosa was recovered from a dry floor 5 weeks after the ward was closed and from burn eschar tissue samples excised 8 weeks earlier (244).
P. aeruginosa was recovered from a dry floor 5 weeks after the ward was closed and from burn eschar tissue samples excised 8 weeks earlier (244).
Acinetobacter demonstrates even better survival than P. aeruginosa under some conditions (240,245, 246, 247, 248, 249 and 250). For example, Acinetobacter calcoaceticus survived an average of 9 days on a dry Formica surface compared with <1 day for P. aeruginosa (247). Survival of A. baumannii at high colony counts for at least 16 weeks on dry surfaces has been shown using a strain initially isolated from dry environmental surfaces (248). In addition, strains of A. baumannii survived on glass coverslips for an average of 27 days when incubated under conditions mimicking the hospital environment (250).
B. cepacia achieved concentrations of 106 to 108 CFU/mL when inoculated into sterile 5% dextrose and normal saline solutions but appeared to exhaust its nutrient supply after 21 days of incubation (251). B. cepacia did not multiply in 50% dextrose, 3% saline, or hyperalimentation solutions (239). After prolonged storage of a contaminated solution of minimal inorganic salts containing benzalkonium chloride and ammonium acetate, cultures demonstrated viable B. cepacia after 14 years (252).
DETECTION AND TYPING
P. aeruginosa grows readily on most standard laboratory media. For isolation from clinical specimens or sources with mixed flora, media that are selective for gram-negative bacilli, such as MacConkey or eosin-methylene blue agars, are utilized (253,254). When culture surveillance of body sites or the environment is warranted, the detection of P. aeruginosa is facilitated by the use of selective media, and agar containing cetyltrimethylammonium bromide (cetrimide) is the most widely employed (255, 256, 257 and 258). Other agents selective for P. aeruginosa include acetamide (259), nitrofurantoin (260), 9-chloro-9-(4-diethylaminophenyl)-10-phenylacridan (C-390) (261, 262 and 263), 1,10-phenanthroline (264), C-390 and phenanthroline (265), and 2,4,4-trichloro-2-hydroxydiphenyl ether (Irgasan) (258). Before embarking on a culture survey with a selective medium, it is worthwhile to first confirm that the chosen medium inhibits competing species but not the strain(s) of interest. After isolation of bacteria on agar, characteristics that distinguish P. aeruginosa include a grapelike odor and production of a bluegreen pigment (pyocyanin). Bacterial colonies exhibiting these features are definitively identified as P. aeruginosa by standard laboratory methods (253,254).
Most nonfermenters also grow well on nonselective media and MacConkey agar (266,267). For patients with cystic fibrosis, the isolation of Burkholderia species from culture is significantly enhanced by the use of selective media. P. cepacia agar and oxidation-fermentation polymyxin-bacitracin-lactose medium increase the yield of B. cepacia to three to four times that of MacConkey agar (268,269) and demonstrate evidence of growth 24 to 48 hours earlier (270). B. cepacia selective agar, containing 1% lactose, 1% sucrose, polymyxin, gentamicin, and vancomycin, achieves better suppression of other respiratory tract pathogens than P. cepacia agar or oxidation-fermentation polymyxin-bacitracin-lactose while allowing the isolation of B. cepacia (271,272). S. maltophilia has been misidentified as B. cepacia based on a false-negative DNase reaction. Because of the important clinical and prognostic implications in patients with cystic fibrosis, careful interpretation of such laboratory assays is essential (273). Selective and differential media for the detection of Acinetobacter species, such as Leeds Acinetobacter medium, Herellea agar, and Holton’s agar, were developed for use with clinical specimens and environmental testing (267,274,275).
Because strain typing systems have become more sophisticated and accessible, epidemiologic investigations of P. aeruginosa are now more efficient and provide increasingly meaningful information. Early typing systems relied on phenotypic characteristics such as biochemical reactions, antibiotic susceptibility patterns, bacteriophage susceptibility, pyocin susceptibility, pyocin production, O serotype, and enzyme electrophoretic mobility. Because of the limited discriminatory power and sometimes cumbersome nature of such methods, these approaches have been largely replaced by DNA-based techniques (276,277). Only serotyping, based on antigenic determinants on cell wall lipopolysaccharide (International Antigenic Typing System), remains a useful, widely available system (276,278,279).
Plasmid profile analysis was among the first nucleic acid-based techniques applied to strain typing. This technique has been used infrequently (280), and its interpretation is limited by the absence of plasmids in some strains and by potential transfer or spontaneous loss of plasmid(s) in others. Newer techniques have focused on chromosomal DNA (genotyping) to demonstrate genetic relatedness. Restriction endonuclease analysis of genomic DNA is the simplest approach and is useful as a screening tool (279,281,282). Ribotyping has been employed successfully in epidemiologic investigations but is the least discriminatory of molecular methods (281,283, 284, 285, 286 and 287). A more precise approach is Southern blot analysis of chromosomal DNA in which restriction endonuclease fragments carrying a specific sequence are detected by a DNA probe. Probes encoding the exotoxin A gene have proven useful (281,283,288, 289, 290 and 291), but not all Pseudomonas strains carry this gene. Probes encoding for phospholipase C or the pilin polypeptide also have been used alone or in combination with exotoxin A gene probes (288,291,292). Restriction endonuclease analysis of genomic DNA using enzymes with infrequent recognition sites followed by pulsed-field gel electrophoresis (PFGE) has proven highly discriminatory in epidemiologic investigations involving P. aeruginosa (255,281,293, 294, 295, 296 and 297). This method is now considered the typing method of choice for P. aeruginosa. P. aeruginosa genotyping is also feasible by polymerase chain reaction (PCR) methodology such as random amplified polymorphic DNA analysis (RAPD) or enterobacterial repetitive intergenic consensus PCR (285,292,298). Multilocus sequence typing (MLST), which involves the phylogenetic analysis of conserved regions of multiple housekeeping genes, is being investigated as a potential tool for the epidemiologic study of Pseudomonas (299).
Similarly, for B. cepacia, the phenotypic typing methods (300) employed in the past are now considered unreliable (154). Ribotyping has documented patient-to-patient transmission (301, 302 and 303); however, PFGE and RAPD are now
the most commonly employed genotypic methods in epidemiologic investigations (154,301, 302, 303, 304, 305 and 306). Genotyping by repetitive extra-palindromic PCR is also reported (307). MLST is emerging as a reliable and discriminatory tool to examine the global molecular epidemiology of B. cepacia (308, 309, 310 and 311).
the most commonly employed genotypic methods in epidemiologic investigations (154,301, 302, 303, 304, 305 and 306). Genotyping by repetitive extra-palindromic PCR is also reported (307). MLST is emerging as a reliable and discriminatory tool to examine the global molecular epidemiology of B. cepacia (308, 309, 310 and 311).
A variety of molecular techniques have been applied to the epidemiologic study of A. baumannii. Plasmid DNA electrophoresis and ribotyping both have limitations and thus largely have been replaced by PFGE or other PCR-based methods (104,107,109,312, 313, 314, 315, 316, 317 and 318). The molecular epidemiology of Acinetobacter, particularly geographically remote clusters, can be undertaken with MLST (319 and 320). Multilocus PCR followed by electron spray ionization mass spectroscopy is a rapid, high-throughput platform for MLST that is not widely available but has been successfully applied to the study of Acinetobacter and correlates with PFGE results (321,322).
For other nonfermenters, older methods such as serotyping (175,323) and plasmid DNA electrophoresis (313,314) are sometimes helpful, and a variety of molecular techniques including ribotyping (324) and PCR-based systems (325, 326, 327, 328, 329, 330, 331, 332 and 333) have been employed. However, PFGE of digested genomic or total DNA is most commonly used for molecular epidemiologic investigations, such as with Stenotrophomonas (147,327,334, 335 and 336) and Chryseobacterium (337,338).
CLINICAL AND EPIDEMIOLOGIC MANIFESTATIONS
Bacteremia
Bacteria may enter the bloodstream either because of microorganism virulence or because direct access is provided by contaminated intravascular devices or fluids. P. aeruginosa bacteremia usually arises by the former mechanism, whereas the latter mechanism accounts for most cases of bacteremia by other nonfermentative gram-negative bacilli.
The frequency of P. aeruginosa bacteremia largely depends on the population of patients studied. National statistics rank P. aeruginosa as the seventh leading cause of bloodstream infection, accounting for 4.3% of all bloodstream infections with an incidence of 2.1 per 10,000 hospitals admissions (155). At university teaching hospitals, the overall incidence has been about 10 cases per 10,000 admissions (339,340). In patients with burn injuries or cancer, the incidence has been about 50 cases per 10,000 admissions (341,342), and the incidence has exceeded 500 cases per 10,000 admissions in patients with acute leukemia (342,343). The great majority of cases of P. aeruginosa bacteremia appears to be healthcare-associated.
The usual clinical picture of P. aeruginosa bacteremia is the same as that of bacteremia caused by other gramnegative bacilli. Fever is almost always present, except in infants, and tachycardia and hypotension are common findings (342,344,345). Necrotizing skin lesions, called ecthyma gangrenosum, are considered pathognomonic of P. aeruginosa bacteremia (346,347), but occasionally are seen in bloodstream infections by other pathogens, including S. maltophilia (83,348,349) and B. cepacia (350). Ecthyma and other skin lesions were not uncommon in infected cancer patients treated in the 1950s and 1960s (351,352), but are rare in later series (342,344,345).
When present in the bloodstream of cancer patients, P. aeruginosa is virtually always the sole pathogen, and bacteremia is thought to arise from the alimentary tract. Gut colonization with P. aeruginosa has been associated with a risk of P. aeruginosa bacteremia exceeding 40% during neutropenia (353, 354 and 355). In other settings, about 20% of the cases of P. aeruginosa bacteremia are polymicrobial (339,344,356). The most common primary sites of infection from which bacteremia arises are the urinary tract and respiratory tract (339,340,344,356).
The outcome of P. aeruginosa bacteremia is poor, especially in neutropenic cancer patients. The mortality rate for these patients was about 90% until the 1970s, when it became common practice to administer combination therapy with gentamicin and carbenicillin presumptively for neutropenic fever (343,351,357). Subsequently, the timely administration of more potent antipseudomonal antibiotics has lowered mortality rates below 40% (358). In unselected patients at teaching hospitals, the mortality rate of P. aeruginosa bacteremia has remained about 40% to 50% (349,359) and exceeds that for other bacteria (360). Some of this mortality can be attributed to the severity of underlying disease in patients with P. aeruginosa bacteremia. A matched cohort study of ICU patients with P. aeruginosa bacteremia reported an overall mortality of 62%, but an attributable mortality of only 15% (361). Improved outcomes have been associated with resolution of neutropenia and the early use of appropriate antibiotics (351,357, 358 and 359,362,363). Poor outcomes have been associated with inappropriate initial antimicrobial therapy (363,364, 365 and 366). Historically, an emphasis was placed on the treatment of P. aeruginosa bacteremia with synergistic antibiotic combinations, typically an antipseudomonal β-lactam agent plus an aminoglycoside. Ongoing analysis suggests that combination therapy is no more effective than monotherapy, provided the single agent is not an aminoglycoside but is an antipseudomonal β-lactam with potent activity given at a suitable dosage (364,365,367). Combination therapy may continue to have value as initial empiric therapy when the identity and the susceptibility profile of the infecting agent are unknown. In striking contrast to the life-threatening nature of most cases of healthcare-associated P. aeruginosa bacteremia, there are occasional examples of asymptomatic P. aeruginosa bacteremia (368) and of symptomatic intravenous catheter sepsis that resolves without specific antibiotic therapy (369,370).
Cases of primary P. aeruginosa bacteremia occasionally have been linked to intravenous devices or infusion products that became contaminated during preparation in hospitals (179,371, 372 and 373). Bacteremia arising from contaminated endoscopes has been a more frequent problem (374,375,376), including episodes reported in association with endoscopic retrograde cholangiopancreatography (377, 378, 379 and 380). Outbreaks of P. aeruginosa infection similarly have been traced to breaches in reprocessing of bronchoscopes (376) and cystoscopes (381). In these cases, the onset of symptoms usually was a few hours to a few days after the procedure. Hemodialysis treatment also has been a source of P. aeruginosa bacteremia and has been associated with inadequate reprocessing of hemodialyzers
with benzalkonium chloride (368,382), incorrectly diluted formaldehyde (383), or contaminated dialysate waste drainage ports (384).
with benzalkonium chloride (368,382), incorrectly diluted formaldehyde (383), or contaminated dialysate waste drainage ports (384).
In some centers treating neutropenic cancer patients, the overall incidence of P. aeruginosa bacteremia has declined (385). However, other centers have reported outbreak or hyperendemic problems apparently linked to contamination of mouthwash (386), environmental contamination (355), or cross-transmission from patients (171).
Pseudobacteremia due to P. aeruginosa contamination of blood culture specimens has been reported (387,388). One outbreak of P. aeruginosa pseudobacteremia was traced to the use of contaminated disinfectant to clean blood culture bottles before use (388) (see also Chapter 9).
Although P. aeruginosa bacteremia is almost always lifethreatening, bacteremia caused by other nonfermenting gram-negative bacilli is frequently self-limited. Outbreaks of B. cepacia complex bacteremia associated with common source exposure to contaminated fluids (89,389, 390, 391, 392 and 393), including alcohol-free mouthwash (394,395), skin moisturizer (396), disinfectants (186,202,397,398), or medical devices (382, 383, 384, 385, 386 and 387,389, 390, 391 and 392,399,400), have been associated with significant morbidity, but there has been little or no mortality. Most reported cases of B. cepacia bacteremia have in common the direct introduction of contaminated material into the bloodstream. Pseudobacteremia due to B. cepacia has been reported rarely (401).
S. maltophilia bacteremia generally arises secondary to respiratory tract or intravenous catheter-related infection in immunocompromised patients receiving broadspectrum antibiotics. S. maltophilia bacteremia often occurs as a breakthrough infection (402). Unlike B. cepacia, S. maltophilia bacteremia is often associated with signs and symptoms of sepsis and carries a mortality rate of 25% to 57% (403, 404 and 405). One case-control study reported an attributable mortality rate of 27% in patients with S. maltophilia bacteremia (406). Mortality is increased when the patient is immunocompromised, the primary source is the lung, or antibiotic therapy is inappropriate (402, 403, 404 and 405,407). Catheter-related bloodstream S. maltophilia infections respond well to early catheter removal; failure to remove the catheter is associated with a high risk of relapse (408,409,410). S. maltophilia has also been associated with outbreaks of pseudobacteremia (411).
Acinetobacter species accounted for 1.5% of all healthcare-associated bloodstream infections in a survey of 49 US hospitals from 1995 to 1998 (412). A. baumannii accounted for 86% of Acinetobacter isolates. A. baumannii bacteremia was more frequently observed in the ICU than bloodstream infections with other gram-negative bacilli (69% vs. 47%, respectively). Acinetobacter bacteremia has the potential to present as very low grade infection or as septic shock. Early reports emphasized the transient or benign nature of Acinetobacter bacteremia (413, 414, 415, 416 and 417). Bacteremia often cleared with removal of the associated intravenous catheter with or without antibiotic therapy. A report of catheter-related Acinetobacter johnsonii bacteremia described a similarly benign clinical course (418). In other series, high fever, leukocytosis, and septic shock were present in 37% to 78% of cases, mortality rates ranged from 15% to 32%, and metastatic complications including endocarditis, septic thrombophlebitis, and intra-abdominal abscess were detected (107,419, 420 and 421). A case-control study of ICU patients with A. baumannii bacteremia found an overall mortality of 42%, but an attributable mortality of only 8%, reflecting the underlying severity of illness in patients with Acinetobacter bacteremia (422). Acinetobacter infection tends to occur in patients with impaired host defenses. Almost all have intravenous catheters and are receiving broad-spectrum antibiotics (107,413, 414, 415, 416, 417, 418, 419, 420 and 421,423,424).
P. fluorescens is an important cause of transfusionassociated infection. This microorganism is psychrophilic (grows at 4°C) and utilizes citrate as a carbon source. Refrigerated citrate anticoagulated red blood cell units serve as an ideal growth medium. P. fluorescens can achieve peak concentrations of 106 to 107 CFU/mL within 1 week of storage at 4°C. Transfusion-related infection has been associated with severe illness and mortality rates exceeding 50% (425, 426 and 427). These infections are characterized by the sudden onset of fever, chills, and hypotension during red blood cell transfusion, and the source of infection has been confirmed by positive culture of untransfused blood. P. fluorescens bacteremia has also been associated with infusion of contaminated heparinized saline flush solution (428). In contrast to the high mortality rate reported with transfusion-related infection, there were no deaths among 80 cases reported, possibly reflecting a lower microorganism load in the infused flushes.
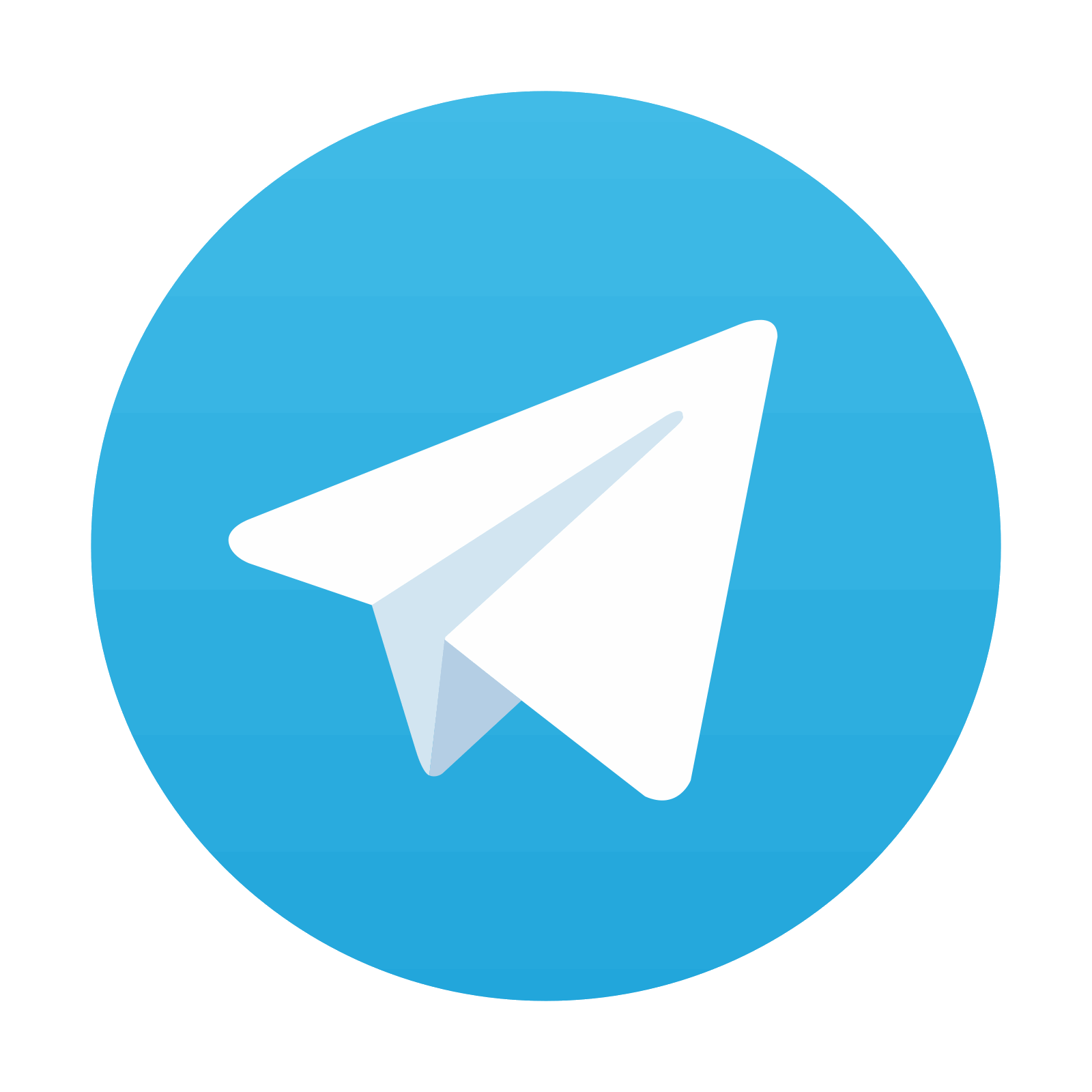
Stay updated, free articles. Join our Telegram channel
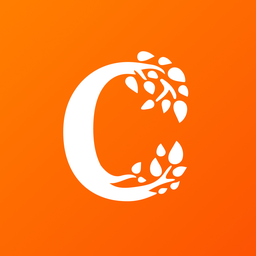
Full access? Get Clinical Tree
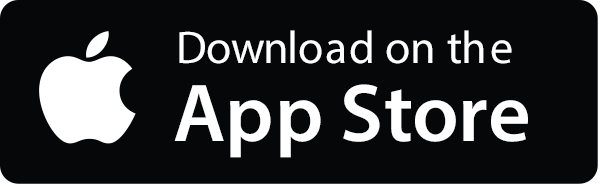
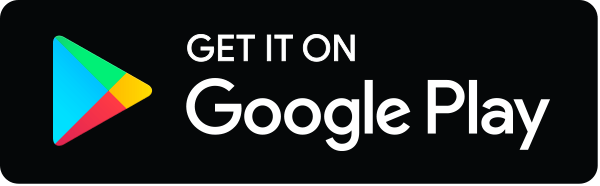