biological systems that would include uses in antimicrobial technologies as well as pharmaceuticals, medical devices, cosmetics, etc. Conversely, items such as electronics use polymers and coatings (including those that are antimicrobial) applied to metals, glass, and plastics, where the nanomaterial is permanently or very strongly bound to the larger molecular structure or are otherwise not generally available for biological contact, the likelihood of significant toxicological effect, and risk is typically expected to be of lesser concern.1
![]() FIGURE 74.1 Nanometer-sized materials dimensions relative to the dimensions of different biological structures and microbes. (Based on Hobson et al.1) |

![]() FIGURE 74.2 Nomenclature based on nanoscale dimensions useful for classification of different types of engineered nanomaterials. (Based on Hobson et al.1) |

mechanisms such as cell wall inhibition and lysis, inhibition of protein synthesis, alteration of cell membranes, inhibition of nucleic acid synthesis, and antimetabolite activity.28 The NP antimicrobials, on the other hand, have a vast array of possible physiochemical properties with respect to size, shape, surface area, surface energy, charge, crystallinity, agglomeration, aggregation, and chemical composition.29,30,31 Although for many NP-antimicrobial materials the precise mechanisms of action are still unknown and are currently under investigation, studies show that NPs often act by causing bacterial cell membrane degradation.32,33,34,35 For example, Li et al35 found that cell membrane degradation of S aureus occurred following exposure to catechin-Cu NPs. Catechin is a crystalline, natural phenol, and antioxidant compound from catechu, the tannic juice or boiled extract of the Mimosa catechu plant (Acacia catechu L.). Catechin-Cu NPs were also found to exert different mechanisms of action that resulted in E coli cell wall degradation. This is thought to be an indication of potentially different impacts on gram-negative and gram-positive bacteria by these NPs.35 Similar types of multiple effects have also been observed with CuNPs that exhibit antimicrobial actions, which, in addition to cell membrane damage, include the generation of reactive oxygen species (ROS) and lipid peroxidation.33
Uptake into cells and transcytosis across cells
Distribution into the blood and lymph circulation to reach potentially sensitive target sites (eg, bone marrow, lymph nodes, spleen, liver, kidneys, and heart)
Brain entry via nasal nerves (eg, polio virus)
Recognition and processing by the immune system
Entry into the cell nucleus
Skin, inhalation and gastrointestinal absorption
A growing list of additional antimicrobial processes
the safety and efficacy of VivaGel®, which is formulated as a mucoadhesive gel and delivered vaginally to relieve the signs and symptoms of bacterial vaginosis (BV) and to reduce risk of recurrence of BV in clinical studies. The gel dendrimer is formulated against human immunodeficiency virus (HIV) and herpes simplex virus and has been reported not to interfere with vaginal or rectal physiological pH.59,60,61,62 The microbicide is meant to disrupt and block viral attachment and/or prevent the viral adsorption from targeting cells of the rectum or vagina and is an example of an innovative nanotechnology-enabled product platform being applied to develop safe and effective new antiviral nanoproducts. These include applications to address a variety of sexual and women’s health concerns including the treatment and prevention of BV, prevention of sexually transmitted infections, and incorporation into condoms.
TABLE 74.1 Examples of different types of engineered nanomaterials and their antimicrobial action | ||||||||||||||||||||||||||||||||||||||||||||||||||||||||||||||||||||||||||||
---|---|---|---|---|---|---|---|---|---|---|---|---|---|---|---|---|---|---|---|---|---|---|---|---|---|---|---|---|---|---|---|---|---|---|---|---|---|---|---|---|---|---|---|---|---|---|---|---|---|---|---|---|---|---|---|---|---|---|---|---|---|---|---|---|---|---|---|---|---|---|---|---|---|---|---|---|
|
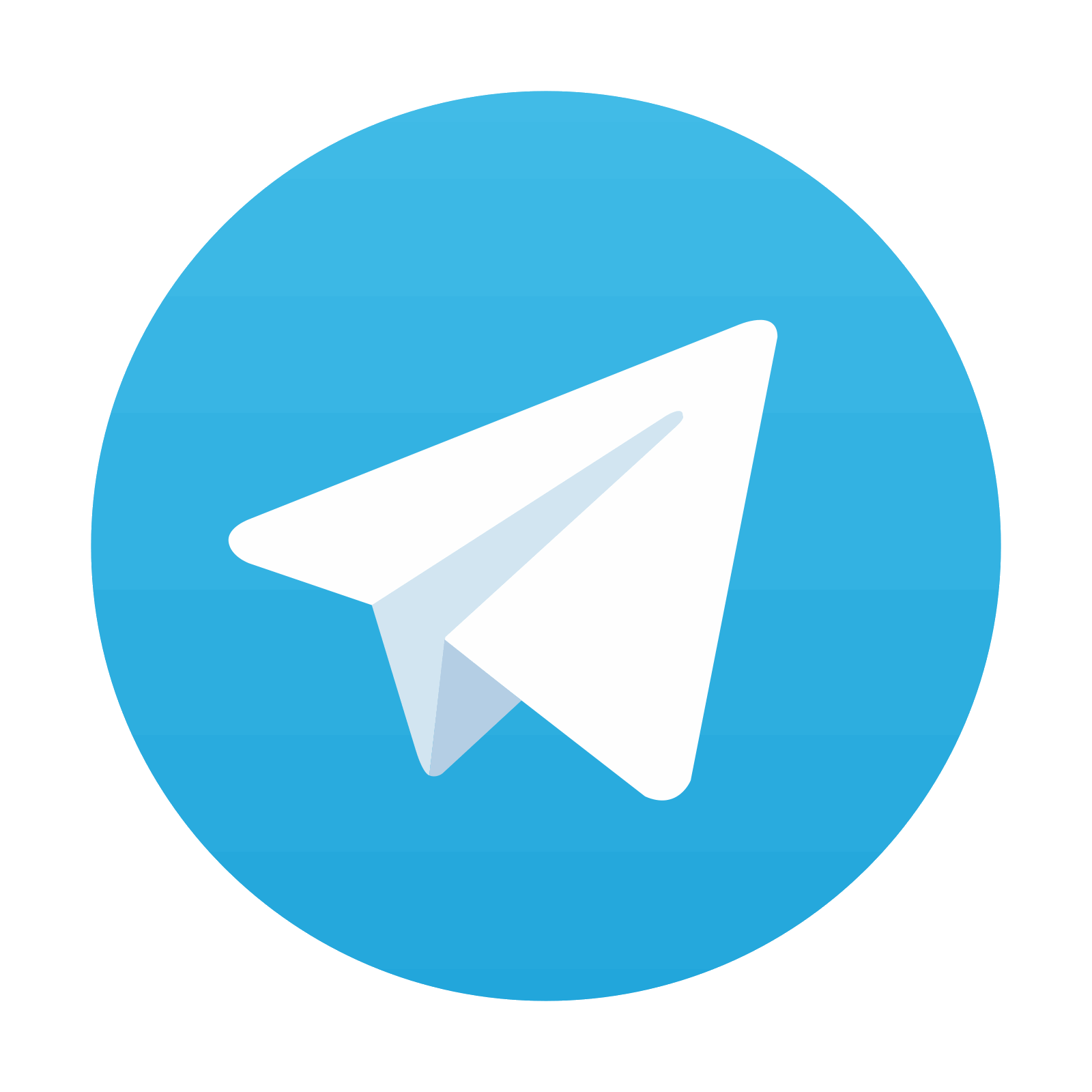
Stay updated, free articles. Join our Telegram channel
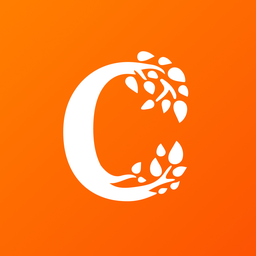
Full access? Get Clinical Tree
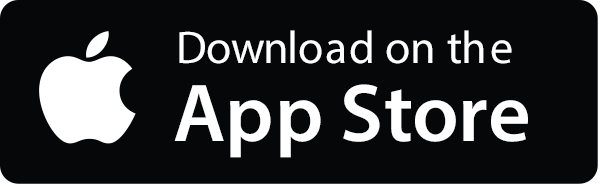
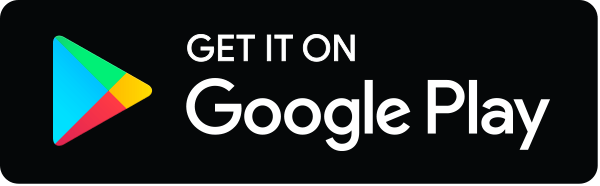