KEY POINTS
When operating on the liver, gallbladder, pancreas, or adjacent organs, recognition of the normal or variant vascular and biliary anatomy is essential to avoiding surgical complications.
The liver is the largest gland in the body and performs a diverse spectrum of functions.
Computed tomography and magnetic resonance imaging with contrast enhancement constitute the mainstays for the radiologic evaluation of the liver.
Acute liver failure rapidly progresses to hepatic coma and death even with maximal medical therapy. The only definitive treatment is orthotopic liver transplantation.
Cirrhosis is the end result of chronic hepatic insult, and further deterioration can lead to the development of end-stage liver disease, which carries a high mortality rate.
Acute variceal bleeding should be managed with aggressive resuscitation and prompt endoscopic diagnosis with hemorrhage control. The transjugular intrahepatic portosystemic shunt procedure can be considered for cases refractory to medical treatment.
Common benign lesions of the liver include cysts, hemangiomas, focal nodular hyperplasia, and hepatocellular adenomas. In most instances, these lesions can be reliably diagnosed by their characteristic features on imaging.
Many options exist for the treatment of hepatocellular carcinomas, and these cases are best managed by a multidisciplinary liver transplant team.
Surgical resection is the treatment of choice for hilar cholangiocarcinoma. Under a protocol with strict eligibility criteria, patients with unresectable tumors can be considered for liver transplantation following neoadjuvant chemoradiation, with survival rates that compare favorably with the rates for resection.
The resectability of colorectal cancer metastases to the liver is primarily determined by the volume of the future liver remnant and the health of the background liver and not actual tumor number.
Laparoscopic liver resections can be performed safely by experienced surgeons in selected patients and have been shown to produce comparable morbidity and mortality rates to open resections.
HISTORY OF LIVER SURGERY
The ancient Greek myth of Prometheus reminds us that the liver is the only organ that regenerates. According to Greek mythology, Zeus was furious with the Titan Prometheus because he gave fire to mortals. In return, Zeus chained Prometheus to Mount Caucasus and sent his giant eagle to eat his liver during the day, only to have it regenerate at night. Although this is folklore, the principles are correct that after hepatic resection, the remnant liver will hypertrophy over weeks to months to regain most of its original liver mass. It is interesting to note that the ancient Greeks seem to have been aware of this fact, because the Greek word for the liver, h¯epar, derives from the verb h¯epaomai, which means “mend” or “repair.” Hence h¯epar roughly translates as “repairable.”1 The importance of the liver dates back to even biblical times, for the Babylonians (c. 2000 B.C.) considered the liver to be the seat of the soul. There are scattered reports of liver surgery for battlefield injuries, but the first recorded elective hepatic resection was done in 1888 in Germany by Langenbuch. There followed reports of liver resections in the United States (Tiffany, 1890) and Europe (Lucke, 1891), as well as the first large series of hepatic resections by Keen in 1899.2,3 In 1908, Pringle described in Annals of Surgery the “arrest of hepatic hemorrhage due to trauma” by compression of the porta hepatis, a maneuver that now bears his name.4 Possibly due to the potential for massive hemorrhage during liver surgery, very little progress in surgical techniques was recorded for the next half-century. Work by Rex, Cantlie, and others laid the groundwork for experimental and clinical reports in the 1950s by Couinaud, Hjortsjo, Healey, Lortat-Jacob, and Starzl.5,6 These seminal contributions paved the way for the modern era of hepatic resection surgery.
LIVER ANATOMY
The liver is the largest organ in the body, weighing approximately 1500 g. It resides in the right upper abdominal cavity beneath the diaphragm and is protected by the rib cage. It is reddish brown and is surrounded by a fibrous sheath known as Glisson’s capsule. The liver is held in place by several ligaments (Fig. 31-1). The round ligament is the remnant of the obliterated umbilical vein and enters the left liver hilum at the front edge of the falciform ligament. The falciform ligament separates the left lateral and left medial segments along the umbilical fissure and anchors the liver to the anterior abdominal wall. Deep in the plane between the caudate lobe and the left lateral segment is the fibrous ligamentum venosum (Arantius’ ligament), which is the obliterated ductus venosus and is covered by the plate of Arantius. The left and right triangular ligaments secure the two sides of the liver to the diaphragm. Extending from the triangular ligaments anteriorly on the liver are the coronary ligaments. The right coronary ligament also extends from the right undersurface of the liver to the peritoneum overlying the right kidney, thereby anchoring the liver to the right retroperitoneum. These ligaments (round, falciform, triangular, and coronary) can be divided in a bloodless plane to fully mobilize the liver to facilitate hepatic resection. Centrally and just to the left of the gallbladder fossa, the liver attaches via the hepatoduodenal and the gastrohepatic ligaments (Fig. 31-2). The hepatoduodenal ligament is known as the porta hepatis and contains the common bile duct, the hepatic artery, and the portal vein. From the right side and deep (dorsal) to the porta hepatis is the foramen of Winslow, also known as the epiploic foramen (see Fig. 31-2). This passage connects directly to the lesser sac and allows complete vascular inflow control to the liver when the hepatoduodenal ligament is clamped using the Pringle maneuver.
The liver is grossly separated into the right and left lobes by the plane from the gallbladder fossa to the inferior vena cava (IVC), known as Cantlie’s line.5 The right lobe typically accounts for 60% to 70% of the liver mass, with the left lobe (and caudate lobe) making up the remainder. The caudate lobe lies to the left and anterior of the IVC and contains three subsegments: the Spiegel lobe, the paracaval portion, and the caudate process.7 The falciform ligament does not separate the right and left lobes, but rather it divides the left lateral segment from the left medial segment. The left lateral and left medial segments also are referred to as sections as defined in the Brisbane 2000 terminology, which is outlined later in the section titled “Hepatic Resection.” A significant advance in our understanding of liver anatomy came from the cast work studies of the French surgeon and anatomist Couinaud in the early 1950s. Couinaud divided the liver into eight segments, numbering them in a clockwise direction beginning with the caudate lobe as segment I.6 Segments II and III comprise the left lateral segment, and segment IV is the left medial segment (Fig. 31-3). Thus, the left lobe is made up of the left lateral segment (Couinaud’s segments II and III) and the left medial segment (segment IV). Segment IV can be subdivided into segment IVA and segment IVB. Segment IVA is cephalad and just below the diaphragm, spanning from segment VIII to the falciform ligament adjacent to segment II. Segment IVB is caudad and adjacent to the gallbladder fossa. Many anatomy textbooks also refer to segment IV as the quadrate lobe. Quadrate lobe is an outdated term, and the preferred term is segment IV or left medial segment. Most surgeons still refer to segment I as the caudate lobe, rather than segment I. The right lobe is comprised of segments V, VI, VII, and VIII, with segments V and VIII making up the right anterior lobe, and segments VI and VII the right posterior lobe.
Additional functional anatomy was highlighted by Bismuth based on the distribution of the hepatic veins. The three hepatic veins run in corresponding scissura (fissures) and divide the liver into four sectors.8 The right hepatic vein runs along the right scissura and separates the right posterolateral sector from the right anterolateral sector. The main scissura contains the middle hepatic vein and separates the right and left livers. The left scissura contains the course of the left hepatic vein and separates the left posterior and left anterior sectors.
The liver has a dual blood supply consisting of the hepatic artery and the portal vein. The hepatic artery delivers approximately 25% of the blood supply, and the portal vein approximately 75%. The hepatic artery arises from the celiac axis (trunk), which gives off the left gastric, splenic, and common hepatic arteries (Fig. 31-4). The common hepatic artery then divides into the gastroduodenal artery and the hepatic artery proper. The right gastric artery typically originates off of the hepatic artery proper, but this is variable. The hepatic artery proper divides into the right and left hepatic arteries. This “classic” or standard arterial anatomy is present in only approximately 76% of cases, with the remaining 24% having variable anatomy. It is critical to understand the arterial (and biliary) anatomic variants to avoid surgical complications when operating on the liver, gallbladder, pancreas, or adjacent organs.
The most common hepatic arterial variants are shown (Fig. 31-5). Approximately 10% to 15% of the time, there is a replaced or accessory right hepatic artery arising from the superior mesenteric artery (SMA). When there is a replacement or accessory right hepatic artery, it travels posterior to the portal vein and then takes up a right lateral position before diving into the liver parenchyma. This can be recognized visually on a preoperative computed tomography (CT) or magnetic resonance imaging (MRI) scan, and confirmed by palpation in the hilum where a separate right posterior pulsation is felt distinct from that of the hepatic artery proper that lies anteriorly in the hepatoduodenal ligament to the left of the common bile duct. In approximately 3% to 10% of cases, there exists a replacement (or accessory) left hepatic artery coming off of the left gastric artery and running obliquely in the gastrohepatic ligament anterior to the caudate lobe before entering the hilar plate at the base of the umbilical fissure. Other less common variants (approximately 1% to 2% each) are the presence of both replaced right and replaced left hepatic arteries, as well as a completely replaced common hepatic artery coming off the SMA (see Fig. 31-5). Although not well demonstrated in the illustration, the clue for a completely replaced common hepatic artery coming off the SMA is the presence of a strong arterial pulsation to the right of and posterior to the common bile duct, rather than the left side and anterior, in the porta hepatis. Another important point is that the right hepatic artery passes deep and posterior to the common bile duct approximately 88% of the time but crosses anterior to the common bile duct in approximately 12% of cases. The cystic artery feeding the gallbladder usually arises from the right hepatic artery in Calot’s triangle.
The portal vein is formed by the confluence of the splenic vein and the superior mesenteric vein. The inferior mesenteric vein usually drains into the splenic vein upstream from the confluence (Fig. 31-6). The main portal vein traverses the porta hepatis before dividing into the left and right portal vein branches. The left portal vein typically branches from the main portal vein outside of the liver with a sharp bend to the left and consists of the transverse portion followed by a 90° turn at the base of the umbilical fissure to become the umbilical portion before entering the liver parenchyma (Fig. 31-7). The left portal vein then divides to give off the segment II and III branches to the left lateral segment, as well as the segment IV branches that supply the left medial segment. The left portal vein also provides the dominant inflow branch to the caudate lobe (although branches can arise from the main and right portal veins also), usually close to the bend between the transverse and umbilical portions. The division of the right portal vein is usually higher in the hilum and may be close to (or inside) the liver parenchyma at the hilar plate. Twenty percent to thirty-five percent of individuals have aberrant portal venous anatomy, with portal vein trifurcation or an aberrant branch from the left portal vein supplying the right anterior lobe being the most frequent.
The portal vein drains the splanchnic blood from the stomach, pancreas, spleen, small intestine, and majority of the colon to the liver before returning to the systemic circulation. The portal vein pressure in an individual with normal physiology is low at 3 to 5 mmHg. The portal vein is valveless, however, and in the setting of portal hypertension, the pressure can be quite high (20 to 30 mmHg). This results in decompression of the systemic circulation through portocaval anastomoses, most commonly via the coronary (left gastric) vein, which produces esophageal and gastric varices with a propensity for major hemorrhage. Another branch of the main portal vein is the superior pancreaticoduodenal vein (which comes off low in an anterior lateral position and is divided during pancreaticoduodenectomy). Closer to the liver, the main portal vein typically gives off a short branch (posterior lateral) to the caudate process on the right side. It is important to identify this branch and ligate it during hilar dissection for anatomic right hemihepatectomy to avoid avulsion.
There are three hepatic veins (right, middle, and left) that pass obliquely through the liver to drain the blood to the suprahepatic IVC and eventually the right atrium (Fig. 31-8). The right hepatic vein drains segments V through VIII; the middle hepatic vein drains segment IV as well as segments V and VIII; and the left hepatic vein drains segments II and III. The caudate lobe is unique because its venous drainage feeds directly into the IVC. In addition, the liver usually has a few small, variable short hepatic veins that directly enter the IVC from the undersurface of the liver. The left and middle hepatic veins form a common trunk approximately 95% of the time before entering the IVC, whereas the right hepatic vein inserts separately (in an oblique orientation) into the IVC. There is a large inferior accessory right hepatic vein in 15% to 20% of cases that runs in the hepatocaval ligament. This can be a source of torrential bleeding if control of it is lost during right hepatectomy. The hepatic vein branches bisect the portal branches inside the liver parenchyma (i.e., the right hepatic vein runs between the right anterior and posterior portal veins; the middle hepatic vein passes between the right anterior and left portal vein; and the left hepatic vein crosses between the segment II and III branches of the left portal vein).
Figure 31-8.
Confluence of the three hepatic veins (HVs) and the inferior vena cava (IVC). Note that the middle and left HVs drain into a common trunk before entering the IVC. a. = artery; v. = vein. (Adapted with permission from Cameron JL, ed. Atlas of Surgery. Vol. I, Gallbladder and Biliary Tract, the Liver, Portasystemic Shunts, the Pancreas. Toronto: BC Decker; 1990:153.)
Within the hepatoduodenal ligament, the common bile duct lies anteriorly and to the right. It gives off the cystic duct to the gallbladder and becomes the common hepatic duct before dividing into the right and left hepatic ducts. In general, the hepatic ducts follow the arterial branching pattern inside the liver. The right anterior hepatic duct usually enters the liver above the hilar plate, whereas the right posterior duct dives behind the right portal vein and can be found on the surface of the caudate process before entering the liver. The left hepatic duct typically has a longer extrahepatic course before giving off segmental branches behind the left portal vein at the base of the umbilical fissure. Considerable variation exists, and in 30% to 40% of cases, there is a nonstandard hepatic duct confluence with accessory or aberrant ducts (Fig. 31-9). The cystic duct itself also has a variable pattern of drainage into the common bile duct. This can lead to potential injury or postoperative bile leakage during cholecystectomy or hepatic resection, and the surgeon needs to expect these variants. The gallbladder sits adherent to hepatic segments IVB (left lobe) and V (right lobe).
Figure 31-9.
Main variations of hepatic duct confluence. As described by Couinaud in 1957, the bifurcation of the hepatic ducts has a variable pattern in approximately 40% of cases. CHD = common hepatic duct; lh = left hepatic; R = right; ra = right anterior; rp = right posterior. (Reproduced with permission from Blumgart LH, Fong Y, eds. Surgery of the Liver and Biliary Tract. 3rd ed, Vol. I. London: Elsevier Science; 2000. Copyright © Elsevier Science.)
The parasympathetic innervation of the liver comes from the left vagus, which gives off the anterior hepatic branch, and the right vagus, which gives off the posterior hepatic branch. The sympathetic innervation involves the greater thoracic splanchnic nerves and the celiac ganglia, although the function of these nerves is poorly understood. The denervated liver after hepatic transplantation seems to function with normal capacity. A common source of referred pain to the right shoulder and scapula as well as the right side or back is the right phrenic nerve, which is stimulated by tumors that stretch Glisson’s capsule or by diaphragmatic irritation.
Lymph is produced within the liver and drains via the perisinusoidal space of Disse and periportal clefts of Mall to larger lymphatics that drain to the hilar cystic duct lymph node (Calot’s triangle node), as well as the common bile duct, hepatic artery, and retropancreatic and celiac lymph nodes. This is particularly important for resection of hilar cholangiocarcinoma, which has a high incidence of lymph node metastases. The hepatic lymph also drains cephalad to the cardiophrenic lymph nodes, and the latter can be pathologically identified on a staging CT or MRI scan.
LIVER PHYSIOLOGY
The liver is the largest gland in the body and has an extraordinary spectrum of functions. These include processes such as storage, metabolism, production, and secretion. One crucial role is the processing of absorbed nutrients through the metabolism of glucose, lipids, and proteins. The liver maintains glucose concentrations in a normal range over both short and long periods by performing several important roles in carbohydrate metabolism. In the fasting state, the liver ensures a sufficient supply of glucose to the central nervous system. The liver can produce glucose by breaking down glycogen through glycogenolysis and by de novo synthesis of glucose through gluconeogenesis from noncarbohydrate precursors such as lactate, amino acids, and glycerol. In the postprandial state, excess circulating glucose is removed by glycogen synthesis or glycolysis and lipogenesis. The liver also plays a central role in lipid metabolism through the formation of bile and the production of cholesterol and fatty acids. Protein metabolism occurs in the liver through amino acid deamination, resulting in the production of ammonia as well as the production of a variety of amino acids. In addition to metabolism, the liver also is responsible for the synthesis of most circulating plasma proteins. Among these proteins are albumin, factors of the coagulation and fibrinolytic systems, and compounds of the complement cascade. Furthermore, the detoxification of many substances through drug metabolism occurs in the liver, as do immunologic responses through the many immune cells found in its reticuloendothelial system.9
Bilirubin is the breakdown product of normal heme catabolism. Bilirubin is bound to albumin in the circulation and sent to the liver. In the liver, it is conjugated to glucuronic acid to form bilirubin diglucuronide in a reaction catalyzed by the enzyme glucuronyl transferase, making it water soluble. This glucuronide is then excreted into the bile canaliculi. A small amount dissolves in the blood and is then excreted in the urine. The majority of conjugated bilirubin is excreted in the intestine as waste, because the intestinal mucosa is relatively impermeable to conjugated bilirubin. However, it is permeable to unconjugated bilirubin and urobilinogens, a series of bilirubin derivatives formed by the action of bacteria. Thus, some of the bilirubin and urobilinogens are reabsorbed in the portal circulation; they are again excreted by the liver or enter the circulation and are excreted in the urine.10
Bile is a complex fluid containing organic and inorganic substances dissolved in an alkaline solution that flows from the liver through the biliary system and into the small intestine. The main components of bile are water, electrolytes, and a variety of organic molecules including bile pigments, bile salts, phospholipids (e.g., lecithin), and cholesterol. The two fundamental roles of bile are to aid in the digestion and absorption of lipids and lipid-soluble vitamins and to eliminate waste products (bilirubin and cholesterol) through secretion into bile and elimination in feces. Bile is produced by hepatocytes and secreted through the biliary system. In between meals, bile is stored in the gallbladder and concentrated through the absorption of water and electrolytes. Upon entry of food into the duodenum, bile is released from the gallbladder to aid in digestion. The human liver can produce about 1 L of bile daily.
Bile salts, in conjunction with phospholipids, are responsible for the digestion and absorption of lipids in the small intestine. Bile salts are sodium and potassium salts of bile acids conjugated to amino acids. The bile acids are derivatives of cholesterol synthesized in hepatocytes. Cholesterol, ingested from the diet or derived from hepatic synthesis, is converted into the bile acids cholic acid and chenodeoxycholic acid. These bile acids are conjugated to either glycine or taurine before secretion into the biliary system. Bacteria in the intestine can remove glycine and taurine from bile salts. They can also convert some of the primary bile acids into secondary bile acids by removing a hydroxyl group, producing deoxycholic acid from cholic acid and lithocholic acid from chenodeoxycholic acid.
Bile salts secreted into the intestine are efficiently reabsorbed and reused. Approximately 90% to 95% of the bile salts are absorbed from the small intestine at the terminal ileum. The remaining 5% to 10% enter the colon and are converted to the secondary salts, deoxycholic acid and lithocholic acid. The mixture of primary and secondary bile salts and bile acids is absorbed primarily by active transport in the terminal ileum. The absorbed bile salts are transported back to the liver in the portal vein and re-excreted in the bile. Those lost in the stool are replaced by synthesis in the liver. The continuous process of secretion of bile salts in the bile, their passage through the intestine, and their subsequent return to the liver is termed the enterohepatic circulation.10
The liver plays an important role in providing mechanisms for ridding the body of foreign molecules (xenobiotics) that are absorbed from the environment. In most cases, a drug is relatively lipophilic to ensure good absorption. The liver participates in the elimination of these lipid-soluble drugs by transforming them into more readily excreted hydrophilic products. There are two main reactions important for drug metabolism. Phase I reactions include oxidation, reduction, and hydrolysis of molecules. These result in metabolites that are more hydrophilic than the original chemicals. The cytochrome P450 system is a family of hemoproteins important for oxidative reactions involving drugs and toxic substances. Phase II reactions, also known as conjugation reactions, are synthetic reactions that involve addition of subgroups to the drug molecule. These subgroups include glucuronate, acetate, glutathione, glycine, sulfate, and methyl groups. These drug reactions occur mainly in the smooth endoplasmic reticulum of hepatocytes.
Many factors can affect drug metabolism in the liver. When the rate of metabolism of a drug is increased (i.e., enzyme induction), the duration of the drug action will decrease. However, when the metabolism of a drug is decreased (i.e., enzyme inhibition), then the drug will circulate for a longer period of time. It is important to note that some drugs may be converted to active products by metabolism in the liver. An example is acetaminophen when taken in larger doses. Normally, acetaminophen is conjugated by the liver to harmless glucuronide and sulfate metabolites that are water soluble and eliminated in the urine. During an overdose, the normal metabolic pathways are overwhelmed, and some of the drug is converted to a reactive and toxic intermediate by the cytochrome P450 system. Glutathione normally reacts with this intermediate, leading to the production and subsequent excretion of a harmless product. However, as glutathione stores are diminished, the reactive intermediate cannot be detoxified and it combines with lipid membranes of hepatocytes, which results in cellular necrosis. Thus, treatment of acetaminophen overdoses consists of replenishing glutathione stores by supplementing with sulfhydryl compounds such as acetylcysteine.
Liver function tests is a term frequently used to refer to measurement of the levels of a group of serum markers for evaluation of liver dysfunction. Most commonly, levels of aspartate transaminase (AST), alanine transaminase (ALT), alkaline phosphatase (AP), γ-glutamyltranspeptidase (GGT), and bilirubin are included in this panel. This term is a misnomer, however, because most of these tests measure not liver function but rather cell damage. More accurate measurement of the liver’s synthetic function is provided by serum albumin levels and prothrombin time (PT). Although measuring liver enzyme levels is important in the assessment of a patient’s liver disease, these test results can be nonspecific. Thus, evaluation of patients with suspected liver disease should always involve careful interpretation of abnormalities in these liver test results in the context of a thorough history and physical examination. The approach to evaluating abnormal laboratory values also can be simplified by categorizing the type of abnormality that predominates (hepatocellular damage, abnormal synthetic function, or cholestasis).
Hepatocellular injury of the liver is usually indicated by abnormalities in levels of the liver aminotransferases AST and ALT. These enzymes participate in gluconeogenesis by catalyzing the transfer of amino groups from aspartic acid or alanine to ketoglutaric acid to produce oxaloacetic acid and pyruvic acid, respectively (these enzymes are also referred to as serum glutamic-oxaloacetic transaminase [SGOT] and serum glutamic-pyruvic transaminase [SGPT]). AST is found in liver, cardiac muscle, skeletal muscle, kidney, brain, pancreas, lungs, and red blood cells and thus is less specific for disorders of the liver. ALT is predominately found in the liver and thus is more specific for liver disease. Hepatocellular injury is the trigger for release of these enzymes into the circulation. Common causes of elevated aminotransferase levels include viral hepatitis, alcohol abuse, medications, genetic disorders (Wilson’s disease, hemochromatosis, α1-antitrypsin deficiency), and autoimmune diseases.
The extent of serum aminotransferase elevations can suggest certain etiologies of the liver injury. However, the levels of the enzymes in these tests correlate poorly with the severity of hepatocellular necrosis, because they may not be significantly elevated in conditions of hepatic fibrosis or cirrhosis. In alcoholic liver disease, an AST:ALT ratio of >2:1 is common. Mild elevations of transaminase levels can be found in nonalcoholic fatty liver disease, chronic viral infection, or medication-induced injury. Moderate increases in the levels of these enzymes are common in acute viral hepatitis. In conditions of ischemic insults, toxin ingestions (i.e., acetaminophen), and fulminant hepatitis, AST and ALT levels can be elevated to the thousands.
Albumin synthesis is an important function of the liver and thus can be measured to evaluate the liver’s synthetic function. The liver produces approximately 10 g of albumin per day. However, albumin levels are dependent on a number of factors such as nutritional status, renal dysfunction, protein-losing enteropathies, and hormonal disturbances. In addition, level of albumin is not a marker of acute hepatic dysfunction due to albumin’s long half-life of 15 to 20 days.
Most clotting factors (except factor VIII) are synthesized exclusively in the liver, and thus their levels can also be used as a measure of hepatic synthetic function. Measurements of the prothrombin time (PT) and international normalized ratio (INR) are some of the best tests of hepatic synthetic function. PT measures the rate of conversion of prothrombin to thrombin. To standardize the reporting of PT and avoid interlaboratory variability, the INR was developed. The INR is the ratio of the patient’s PT to the mean control PT. Because vitamin K is involved in the γ-carboxylation of factors used to measure PT (factors II, VII, IX, and X), values also may be prolonged in other conditions such as vitamin K deficiency and warfarin therapy.
Cholestasis is a condition in which bile flow from the liver to the duodenum is impaired. Disturbances in bile flow may be due to intrahepatic causes (hepatocellular dysfunction) or extrahepatic causes (biliary tree obstruction). Cholestasis often results in the release of certain enzymes and thus can be detected by measuring the serum levels of bilirubin, AP, and GGT. Bilirubin is a breakdown product of hemoglobin metabolism. Unconjugated bilirubin is insoluble and thus is transported to the liver bound to albumin. In the liver, it is conjugated to allow excretion in bile. Measured total bilirubin levels can be normal or high in patients with significant liver disease because of the liver’s ability to conjugate significant amounts of bilirubin. Thus, to help aid in the diagnosis of hyperbilirubinemia, fractionation of total bilirubin is usually performed to distinguish between conjugated (direct) and unconjugated (indirect) bilirubin. Indirect bilirubin is a term frequently used to refer to unconjugated bilirubin in the circulation because the addition of another chemical is necessary to differentiate this fraction from the whole. Normally, >90% of serum bilirubin is unconjugated. The testing process for conjugated bilirubin, in contrast, is direct without the addition of other agents. The direct bilirubin test measures the levels of conjugated bilirubin and δ bilirubin (conjugated bilirubin bound to albumin).
The patterns of elevation of the different fractions of bilirubin provide important diagnostic clues as to the cause of cholestasis. In general, an elevated indirect bilirubin level suggests intrahepatic cholestasis, and an elevated direct bilirubin level suggests extrahepatic obstruction. Mechanisms that can result in increases in unconjugated bilirubin levels include increased bilirubin production (hemolytic disorders and resorption of hematomas) or defects (inherited or acquired) in hepatic uptake or conjugation. The rate-limiting step in bilirubin metabolism is the excretion of bilirubin from hepatocytes, so conjugated hyperbilirubinemia can be seen in inherited or acquired disorders of intrahepatic excretion or extrahepatic obstruction. Conjugated bilirubin that cannot be excreted accumulates in hepatocytes, which results in its secretion into the circulation. Because conjugated bilirubin is water soluble, it can be found in the urine of patients with jaundice.
AP is an enzyme with a wide tissue distribution but is found primarily in the liver and bones. In the liver, it is expressed by the bile duct epithelium. In conditions of biliary obstruction, levels rise as a result of increased synthesis and release into the serum. Because the half-life of serum AP is approximately 7 days, it may take several days for levels to normalize even after resolution of the biliary obstruction.
GGT is another enzyme found in hepatocytes and released from the bile duct epithelium. Elevation of GGT is an early marker and also a sensitive test for hepatobiliary disease. Like AP elevation, however, it is nonspecific and can be produced by a variety of disorders in the absence of liver disease. Increased levels of GGT can be induced by certain medications, alcohol abuse, pancreatic disease, myocardial infarction, renal failure, and obstructive pulmonary disease. For this reason, elevated GGT levels are often interpreted in conjunction with other enzyme abnormalities. For example, a raised GGT level with increased AP level supports a liver source.
Jaundice refers to the yellowish staining of the skin, sclera, and mucous membranes with the pigment bilirubin. Hyperbilirubinemia usually is detectable as jaundice when blood levels rise above 2.5 to 3 mg/dL. Jaundice can be caused by a wide range of benign and malignant disorders. However, when present, it may indicate a serious condition, and thus knowledge of the differential diagnosis of jaundice and a systematic approach to the workup of the patient is necessary. Workup of a patient with jaundice is simplified by organizing the possible causes of the disorder into groups based on the location of bilirubin metabolism. As mentioned previously, bilirubin metabolism can take place in three phases: prehepatic, intrahepatic, and posthepatic. The prehepatic phase includes the production of bilirubin from the breakdown of heme products and its transport to the liver. The majority of the heme results from red blood cell metabolism and the rest from other heme-containing organic compounds such as myoglobin and cytochromes. In the liver, the insoluble unconjugated bilirubin is then conjugated to glucuronic acid to allow for solubility in bile and excretion. The posthepatic phase of bilirubin metabolism consists of excretion of soluble bilirubin through the biliary system into the duodenum. Dysfunction in any of these phases can lead to jaundice.10
Jaundice as a result of elevated levels of unconjugated bilirubin occurs from faulty prehepatic metabolism and usually arises from conditions that interfere with proper conjugation of bilirubin in the hepatocyte. Insufficient conjugation is often seen in processes that result in excessive heme metabolism. Subsequently, the conjugation system is overwhelmed, which results in unconjugated hyperbilirubinemia. Causes of hemolysis include inherited and acquired hemolytic anemias. Inherited hemolytic anemias include genetic disorders of the red blood cell membrane (hereditary spherocytosis and eliptocytosis), enzyme defects (glucose-6-phosphate dehydrogenase deficiency), and defects in hemoglobin structure (sickle cell anemia and thalassemias). Hemolytic anemias can also be acquired, and these can be further divided into those with immune-mediated and those with non–immune-mediated causes. Immune-mediated hemolytic anemias result in a positive finding on a direct Coombs’ test and have a variety of autoimmune and drug-induced causes. In contrast, direct Coombs’ test results are negative in nonimmune hemolytic anemias. The causes in this latter category are varied and include drugs and toxins that directly damage red blood cells, mechanical trauma (heart valves), microangiopathy, and infections. Prehepatic dysfunction of bilirubin metabolism also can result from failure in the transport of unconjugated bilirubin to the liver by albumin in any condition that leads to plasma protein loss. A poor nutritional state or excess protein loss as seen in burn patients can lead to elevated levels of unconjugated bilirubin in the circulation and jaundice.
Intrahepatic causes of jaundice involve the intracellular mechanisms for conjugation and excretion of bile from the hepatocyte. The enzymatic processes in hepatocytes can be affected by any condition that impairs hepatic blood flow and subsequent function of the liver (ischemic or hypoxic events). Furthermore, there are multiple inherited disorders of enzyme metabolism that can result in either unconjugated or conjugated hyperbilirubinemia. Gilbert’s syndrome is a genetic variant characterized by diminished activity of the enzyme glucuronyltransferase, which results in decreased conjugation of bilirubin to glucuronide. It is a benign condition that affects approximately 4% to 7% of the population. Typically, the disease results in transient mild increases in unconjugated bilirubin levels and jaundice during episodes of fasting, stress, or illness. These episodes are self-limited and usually do not require further treatment. Another inherited disorder of bilirubin conjugation is Crigler-Najjar syndrome. It is a rare disease found in neonates and can result in neurotoxic sequelae from bilirubin encephalopathy.
In addition to defects in conjugation, disorders in bilirubin excretion in hepatocytes can also lead to jaundice. Rotor’s syndrome and Dubin-Johnson syndrome are two uncommon genetic disorders that disrupt secretion of conjugated bilirubin from the hepatocyte into the bile and result in conjugated hyperbilirubinemia. There are also multiple acquired conditions that result in inflammation and intrahepatic cholestasis by affecting hepatocyte mechanisms for conjugation and excretion of bile. Viruses, alcohol abuse, sepsis, and autoimmune disorders all can result in inflammation in the liver with subsequent disruption of bilirubin transport in the liver. In addition, jaundice can also occur from the cytotoxic effects of many medications, including acetaminophen, oral contraceptives, and anabolic steroids.
Posthepatic causes of jaundice are usually the result of intrinsic or extrinsic obstruction of the biliary duct system that prevents the flow of bile into the duodenum. There is a wide spectrum of pathologies that may present with obstructive jaundice. Intrinsic obstruction can occur from biliary diseases, including cholelithiasis, choledocholithiasis, benign and malignant biliary strictures, cholangiocarcinoma, cholangitis, and disorders of the papilla of Vater. Extrinsic compression of the biliary tree is commonly due to pancreatic disorders. Patients with pancreatitis, pseudocysts, and malignancies can present with jaundice due to external compression of the biliary system. Finally, with the growing armamentarium of endoscopic tools and minimally invasive surgical approaches, surgical complications are becoming more frequent causes of extrahepatic cholestasis. Misadventures with surgical clips, retained stones, and inadvertent ischemic insults to the biliary system can result in obstructive jaundice recognized at any time from immediately postoperatively to many years later.
RADIOLOGIC EVALUATION OF THE LIVER
Abdominal ultrasound is a commonly applied imaging modality used to evaluate abdominal symptoms. Ultrasound technology is based on the pulse-echo principle. The ultrasound transducer converts electrical energy to high-frequency sound energy that is transmitted into tissue. Although some of the ultrasound waves are transmitted through the tissue, some are reflected back, and the ultrasound image is produced when the ultrasound receiver detects those reflected waves. This real-time gray scale (B-mode) imaging is augmented by Doppler flow imaging. Doppler ultrasound not only can detect the presence of blood vessels but also can determine the direction and velocity of blood flow. Ultrasonography is a useful initial imaging test of the liver because it is inexpensive, widely available, involves no radiation exposure, and is well tolerated by patients. It is excellent for diagnosing biliary pathology and focal liver lesions. In addition, liver injury can be evaluated in trauma patients using the focused abdominal sonography for trauma examination. Limitations of ultrasound include incomplete imaging of the liver, most often at the dome or beneath ribs on the surface, and incomplete visualization of lesion boundaries. Moreover, obesity and overlying bowel gas also can interfere with image quality. Thus, ultrasonographically detected masses usually require further evaluation by other imaging modalities due to the lower sensitivity and specificity of ultrasound compared with CT and MRI.
The advent of contrast-enhanced ultrasound has improved the ability of this modality to differentiate among benign and malignant lesions. The injection of gas microbubble agents can increase the sensitivity and specificity of ultrasound in detecting and diagnosing liver lesions. Microbubbles are <10 μm and, when given intravenously, allow for more effective echo enhancement. Contrast-enhanced ultrasound imaging of the liver improves delineation of liver lesions through identification of dynamic enhancement patterns and the vascular morphology of the lesion. In addition, some agents exhibit a late liver-specific phase in which the bubbles are taken up by cells in the reticuloendothelial system and accumulate in normal liver parenchyma after the vascular enhancement has faded.
The use of intraoperative ultrasound of the liver has rapidly expanded over the years with the increasing number and complexity of hepatic resections being performed.11 It has the ability to provide the surgeon with real-time accurate information useful for surgical planning. Intraoperative ultrasound is considered the gold standard for detecting liver lesions, and studies have shown that it can identify 20% to 30% more lesions than other preoperative imaging modalities. Importantly, it has been shown to influence surgical management in almost 50% of planned liver resections for malignancies. Applications for intraoperative ultrasound of the liver include tumor staging, visualization of intrahepatic vascular structures (Fig. 31-10), and guidance of resection plane by assessment of the relationship of a mass to the vessels. In addition, biopsy of lesions and ablation of tumors can be guided by intraoperative ultrasound.
Figure 31-10.
Intraoperative liver ultrasound images of the portal veins, hepatic veins, and inferior vena cava (IVC). Upper panel shows the portal vein bifurcation with echogenic Glissonian sheath. The confluence of the three hepatic veins (right hepatic vein [RHV], middle hepatic vein [MHV], and left hepatic vein [LHV]) and the IVC is shown in the middle panel. An accessory LHV is present in this patient. Lower panel is a color Doppler image showing flow.
Ultrasound elastography, also referred to as transient elastography, can be used to assess the degree of fibrosis or cirrhosis in the liver. Low-frequency vibrations transmitted through the liver induce an elastic shear wave that is detected by pulse-echo ultrasonography as the wave propagates through the liver. The velocity of the wave correlates with the stiffness of the organ—the wave travels faster through fibrotic or cirrhotic tissues. Ultrasound elastography has been found in large cohorts of individuals to have a sensitivity of 87% and a specificity of 91% for the diagnosis of cirrhosis when compared with liver biopsy.12 Unlike liver biopsy, ultrasound elastography is noninvasive and can be repeated often without additional risk to the patient. Furthermore, this rapid test can acquire information from a larger area of the tissue relative to needle biopsy, providing a better understanding of the entire hepatic parenchyma and reducing sampling error.
CT produces a digitally processed cross-sectional image of the body from a large series of x-ray images. The introduction of helical (spiral) CT has improved the imaging capabilities of this technique compared to earlier conventional axial CT by combining a continuous patient-table motion with continuous rotation of the CT gantry and allowing rapid acquisition of a volume of data within a single breath hold. With the recent advent of multi-detector row CT scanners, high-resolution images can be obtained in submillimeter section thickness within a short scan time, with virtually no penalty in increased radiation dose. Together, these technologic advances have led to reduced motion artifacts due to variations in inspiration, facilitated optimal contrast delivery, and allowed for the capability to generate high-resolution reformations in any desired plane. In a single examination, modern-day CT scans provide detailed morphologic information on the number, size, distribution, and vascularity of liver lesions, all of which are vital in guiding the clinical management and therapeutic plan.
Contrast medium is routinely used in CT evaluation of the liver because of the similar densities of most pathologic liver masses and normal hepatic parenchyma. A CT scan with a dual- or triple-phase bolus of intravenous contrast agent is performed to achieve the greatest enhancement of contrast between normal and pathologic tissues.13 Ideally, contrast media should be selectively delivered to either the tumor or the liver, but not both. Radiologists use the dual blood supply of the liver and the hemodynamics of hepatic tumors to achieve this goal. The liver is unique in that it has a dual blood supply. As previously noted, the portal vein supplies approximately 75% of the blood flow and the hepatic artery the remaining 25%. However, many liver tumors receive the majority of their blood supply from the hepatic artery. After injection of the contrast agent, the rapid scan time of helical CT allows for CT sections through the liver in both the arterial dominant phase (20 to 30 seconds after the beginning of contrast delivery) and venous or portal dominant phase (60 to 70 seconds after contrast injection) (Fig. 31-11). Thus, many hepatic tumors that derive the majority of their blood supply from the hepatic artery as well as other hypervascular lesions are well delineated in the arterial phase. On the other hand, the portal phase provides optimal enhancement of the normal liver parenchyma because the majority of its blood supply is derived from the portal vein. This allows for detection of hypovascular lesions because they will appear hypoattenuated in relation to the brighter normal liver parenchyma.3 Furthermore, the arterial and portal phase images allow for noninvasive mapping of the hepatic arterial and venous anatomy, information that is crucial in the preoperative planning for patients undergoing liver surgery.
Figure 31-11.
Computed tomographic (CT) images of hepatic veins and Couinaud’s liver segments. The images show the three hepatic veins and inferior vena cava (IVC) (upper panel), as well as Couinaud’s liver segments (lower panels). LHV = left hepatic vein; MHV = middle hepatic vein; RHV = right hepatic vein.
CT cholangiography has emerged as a new imaging modality for biliary disease. This technique usually involves the use of contrast agents, which are excreted by hepatocytes into the bile ducts. CT cholangiography, therefore, provides information on hepatocyte function and bile flow, in addition to high-resolution depiction of the biliary tree. It has compared well to endoscopic retrograde cholangiopancreatography (ERCP) in identifying obstructive biliary disease.14 One of the major advantages of CT cholangiography over other imaging modalities is the ability to depict small nondilated peripheral biliary radicals. This technique may be useful in the context of live liver donation or complex biliary surgery to aid in the preoperative depiction of biliary anatomy. It also may be applicable in the postoperative setting for the detection of biliary leakage or obstruction. A limitation of CT cholangiography is that the biliary tree may not be well visualized in patients with excessively dilated bile ducts or in those with hyperbilirubinemia, as bilirubin excretion is impaired in these cases.
MRI is a technique that produces images based on magnetic fields and radio waves. The MRI scanner creates a powerful magnetic field that aligns the hydrogen atoms in the body, and radio waves are used to alter the alignment of this magnetization. Different tissues absorb and release radio wave energy at different rates, and this information is used to construct an image of the body. Most tissues can be differentiated by differences in their characteristic T1 and T2 relaxation times. T1 is a measure of how quickly a tissue can become magnetized, and T2 measures how quickly it loses its magnetization. As with CT technology, advances in MRI now provide the opportunity to perform single-breath T1-weighted imaging and respiration-triggered T2-weighted imaging. The development of breath-hold imaging techniques has eliminated many of the motion artifacts that previously limited the sensitivity and application of MRI for imaging of the liver. Compared with CT scanning, the major advantages of MRI pertain to higher soft tissue contrast resolution and excellent depiction of fluid-containing structures, while obviating the need for ionizing radiation.
As with the iodinated contrast media use in CT scanning, multiple contrast agents have been developed for MRI to increase the difference in signal intensity between normal liver and pathologic lesions. Various gadolinium-based compounds have been used as MRI contrast agents that behave in a manner very similar to iodine in CT. Liver-specific MRI contrast agents also have been developed that rely either on excretion by Kupffer cells, such as ferumoxide (Feridex, Advanced Magnetics, Cambridge, MA), or on secretion in bile by hepatocytes, including gadoxetate (Eovist or Primovist, Bayer-Schering, Berlin, Germany). These agents combine the information obtained during a standard MRI with additional functional data, which in turn yields improved detection and characterization of lesions within the liver.15
Just as ultrasound elastography is useful in the diagnosis of hepatic fibrosis and cirrhosis, magnetic resonance (MR) elastography appears promising as an imaging modality in reducing the need for liver biopsy. In this technique, a vibration device is used to induce a shear wave in the liver. A modified MRI machine detects the shear wave, then generates a color-coded image that depicts the wave velocity, and hence stiffness, throughout the organ. Although preliminary studies have shown that MR elastography can detect cirrhosis with a high degree of accuracy, the clinical utility of this modality, especially given its relatively high cost, remains to be determined.
Magnetic resonance cholangiopancreatography (MRCP) enables rapid, noninvasive depiction of both the biliary tree and the pancreatic duct without the use of ionizing radiation or intravenous contrast media. One of the most common clinical indications for MRCP is biliary obstruction. MRCP provides visualization of dilated bile ducts, and the high spatial and contrast resolution often enables accurate assessment of the level of occlusion in the biliary tree. MRCP also can be enhanced with liver-specific MRI contrast agents that are actively secreted into the bile, but the clinical indications for such studies are still a matter of intensive investigation.16
Positron emission tomography (PET) is a nuclear medicine test that produces images of metabolic activity in tissues by detecting gamma rays emitted by a radioisotope incorporated into a metabolically active molecule. Fluorodeoxyglucose (FDG) is the most common metabolic molecule used in PET imaging. Although traditional imaging such as CT, ultrasound, and MRI provide anatomic information, PET offers functional imaging of tissues with high metabolic activity, including most types of metastatic tumors. PET imaging increasingly is used as a tool in the diagnostic evaluation of a patient with potentially resectable hepatic disease. In nonrandomized trials, PET demonstrated better sensitivity and specificity than CT scanning for the detection of both intrahepatic and extrahepatic disease.17 Integrated PET/CT improves diagnostic accuracy over standard PET or CT alone and has been shown to be sensitive in the detection of liver metastases derived from a wide range of cancers, including colorectal (Fig. 31-12), breast, or lung primaries.17
Figure 31-12.
Computed tomography (CT)–positron emission tomography (PET) scans before and after resection of liver metastasis from colorectal cancer in a 54-year-old patient. CT scan shows large 10-cm right lobe liver metastasis (left panel), and PET scan findings are strongly positive (middle panel). Two years after right hepatectomy, the patient has no evidence of recurrence and significant hypertrophy of the left lobe (right panel).
More than 20% of patients with colorectal cancer initially present with hepatic metastasis, and a large percentage of patients undergoing resection for their primary colorectal cancer eventually experience disease recurrence in the liver. The role of FDG-PET/CT in colorectal cancers lies predominantly in tumor staging and follow-up, particularly in the detection of occult intrahepatic metastases or extrahepatic disease. Although hepatic resection of colorectal metastases provides survival rates nearing 50%, the presence of extrahepatic disease is a poor prognosticator and usually precludes aggressive surgical intervention. Thus, accurate information regarding the extent of the disease is necessary for management of patients with colorectal metastases. PET/CT has also been shown to be more accurate than contrast-enhanced CT in tumor surveillance after radiofrequency ablation.18 The sensitivity of FDG-PET, however, is lowered by neoadjuvant chemotherapy, most likely secondary to reduced metabolic activity within the tumor.
Although the role of PET/CT in the clinical management of liver metastases has been well-established, its utility in the diagnostic workup of primary liver tumors is still debated. In hepatocellular carcinoma (HCC), FDG uptake correlates with the degree of differentiation—high-grade HCC lesions have increased FDG uptake compared to low-grade HCCs. As a result, the overall sensitivity of FDG-PET/CT in the detection of HCCs is reported to be only 50% to 65%, rendering this modality insufficient when used alone in the diagnosis of primary HCCs. For this reason, dual-tracer PET has been introduced to improve sensitivity in detecting all HCC. This modality combines the use of FDG, which accumulates in poorly differentiated tumors, with 11C-acetate, a tracer preferentially accumulated by well-differentiated HCC lesions. Although the clinical benefits of dual-tracer PET/CT have yet to be fully established, this combined modality has the potential to become a valuable tool in the diagnosis and staging of HCC. In cholangiocarcinoma tumors, FDG avidity depends on the morphologic characteristics and location of the lesion. Therefore, FDG-PET and FDG-PET/CT have not been shown to be highly beneficial in the diagnosis of primary cholangiocarcinoma, but they may be beneficial in the detection of regional and distal metastases, which can affect clinical decision making and patient management.
ACUTE LIVER FAILURE
Acute liver failure (ALF) occurs when the rate and extent of hepatocyte death exceeds the liver’s regenerative capabilities. It was initially described as a specific disease entity in the 1950s. It also has been referred to as fulminant hepatic failure. ALF is a rare disorder affecting approximately 2000 patients annually in the United States. ALF is defined by the development of hepatic encephalopathy occurring within 26 weeks of severe liver injury in a patient without a history of previous liver disease or portal hypertension.19 The manifestations of ALF may include cerebral edema, hemodynamic instability, increased susceptibility to bacterial and fungal infections, renal failure, coagulopathy, and metabolic disturbances. Even with current medical care, ALF can progress rapidly to hepatic coma and death. The most common cause of death is intracranial hypertension due to cerebral edema, followed by sepsis and multisystem organ failure. The causes of ALF, which are the most important variables in determining outcome, are numerous and can include viral infection as well as drug overdose, reaction, and toxicity. It has been determined that the etiologic factor leading to ALF varies according to geographic location.20 Before the introduction of orthotopic liver transplantation (OLT), the chance for survival was <20%. Currently, most series report 5-year survival rates of >70% for affected patients.21
Differences in etiology, management, and patient outcomes have been described for various regions of the globe. In the East and developing portions of the world, the most common causes of ALF are viral infections, primarily hepatitis B, A, and E.20 In these areas, there are a relatively small number of drug-induced cases. In contrast, 65% of cases of ALF in the West are thought to be due to drugs and toxins, with acetaminophen (paracetamol) being the most common etiologic agent in the United States, Australia, United Kingdom, and most of Europe. In France and Spain, where acetaminophen sales are restricted, the rate of acetaminophen-induced ALF is quite low.22 Acetaminophen-induced ALF is also uncommon in South America. The U.S. Acute Liver Failure Study Group identified several other causes of ALF, including autoimmune hepatitis, hypoperfusion of the liver (in cardiomyopathy or cardiogenic shock), pregnancy-related conditions, and Wilson’s disease.23 Even with exhaustive efforts to identify a cause, approximately 20% of all cases of ALF remain indeterminate in origin.
In a multicenter study involving 17 tertiary care centers and 308 patients in the United States, 73% of all patients with ALF were female, with a median age of 38 years.24 The most common ethnic group affected was whites (74%), followed by Hispanics (9%) and African Americans (3%). Patients were ill for a median of 6 days before the onset of encephalopathy and had a median of 2 days between the onset of jaundice and the development of encephalopathy. Hepatic coma grade at presentation was approximately equally distributed across grades I to IV. Eighty-four percent of the patients in the study were referred from outside hospitals, 40% had a serum creatinine level exceeding 2.0 mg/dL, and 14% had an arterial pH of <7.30. In addition, 44% of the patients acquired a culture-proven infection.
When the medical history is obtained, it is important to address the possibility of exposure to viral infections, medications, and other possible toxins. The possibility of previous liver disease needs to be explored. The physical examination must assess and document the patient’s mental status as well as attempt to identify findings of chronic liver disease. The initial laboratory examination must evaluate the severity of the ALF as well as attempt to identify the cause (Table 31-1). A liver biopsy should be performed if certain disease entities such as autoimmune hepatitis or lymphoma are a possibility. Because of the associated coagulopathy, if a liver biopsy is needed, it is usually safest to obtain the tissue via a transjugular approach. Patients with ALF should be admitted to the hospital and monitored frequently. Due to the rapidity with which this disease process may progress, a liver transplant center should be contacted and the affected patient transferred to the center early in the evaluation period.
Complete blood count Complete metabolic panel Amylase and lipase levels Liver function tests Prothrombin time/international normalized ratio Factor V level Factor VII level Arterial blood gas concentrations Arterial serum ammonia level ABO typing Acute hepatitis panel Autoimmune marker levels Ceruloplasmin level Toxicology screening Acetaminophen level HIV screening Pregnancy test (females) |
If acetaminophen overdose is suspected to have occurred within a few hours of presentation, administration of activated charcoal may be useful to reduce the volume of acetaminophen present in the gastrointestinal (GI) tract. N-acetylcysteine (NAC), the clinically effective antidote for acetaminophen overdose, should be administered as early as possible to any patient with suspected acetaminophen-associated ALF.25 NAC also should be administered to patients with ALF of unclear etiology, because replenishing glutathione may be beneficial in this patient population as well.26 NAC can be administered either orally (140 mg/kg initial dose, followed by 70 mg/kg every 4 hours × 17 doses) or via the intravenous route (loading dose of 150 mg/kg, followed by a maintenance dose of 50 mg/kg every 4 hours × 12 doses). For patients who are suspected of having drug-induced hepatotoxicity, it is important to obtain details regarding all prescription and nonprescription drugs, herbs, and dietary supplements that may have been taken in the previous year. Most instances of drug-induced hepatotoxicity occur in the first 6 months after drug initiation. Any suspected offending agent must be discontinued, and an attempt should be made to administer only essential medications.
The majority of patients with ALF need to be monitored in the intensive care unit (ICU) setting, and specific attention needs to be given to fluid management, ulcer prophylaxis, hemodynamic monitoring, electrolyte management, and surveillance for and treatment of infection. Surveillance cultures should be performed to identify bacterial and fungal infections as early as possible. Serum phosphorus levels need to be monitored. Hypophosphatemia, a sign of hepatic regeneration, may indicate a higher likelihood of spontaneous recovery and needs to be corrected via intravenous (IV) administration of phosphate. Sedation should be avoided, and the head of the bed should be elevated at least 30°. Neurologic examinations should be performed frequently. Intracranial pressure monitoring is reserved for patients in whom a neurologic examination is no longer reliable. CT scans of the head should be performed to rule out mass lesion or hemorrhage, but they provide only limited information regarding increased intracranial pressure. The administration of blood products for thrombocytopenia and prolonged PT is recommended only in the setting of hemorrhage or before invasive procedures. Acute renal failure is a frequent complication in patients with ALF, and efforts should be made to protect renal function by maintaining sufficient perfusion and avoiding nephrotoxic medications. Should renal replacement therapy become necessary, continuous venovenous hemodialysis should be used rather than intermittent hemodialysis, because continuous venovenous hemodialysis provides better hemodynamic and intracranial pressure stability. The most severely affected patients have a poor prognosis with medical management alone and require liver transplantation. Identifying these patients early in the clinical course is important both to maximize the time available to obtain a donor liver allograft for those in need and to avoid transplant in those who will recover without it.
Accurate identification of ALF patients who will recover spontaneously is important because of the severe shortage of donor liver allografts and the potential complications of lifelong nonspecific immunosuppression. The most widely applied prognostic scoring system is the King’s College Hospital ALF criteria.27 This scoring system has separate criteria predicting a poor medical management outcome for acetaminophen-related and non–acetaminophen-related forms of ALF (Table 31-2). Many other prognostic models exist such as the Acute Physiology and Chronic Health Evaluation II (APACHE II) score, the Clichy criteria,28 and actin-free Gc-globulin serum concentration.29 Overall, prognostic scoring systems have proven to have acceptable specificity but low sensitivity in determining patient outcome and therefore should not replace the judgment of an experienced clinician.30
CAUSE | SELECTION CRITERIA |
---|---|
Acetaminophen | Arterial pH <7.30 irrespective of hepatic coma grade Or Prothrombin time >100 s + serum creatinine level >3.4 mg/dL + grade III or IV hepatic coma |
Not acetaminophen | Prothrombin time >100 s irrespective of hepatic coma grade Or Any three of the following, irrespective of hepatic coma grade: Cryptogenic or drug-induced hepatitis Jaundice to coma interval >7 d Prothrombin time >50 s Serum bilirubin level >17.5 mg/dL Age <10 y or >40 y |
Despite advances in medical management, OLT remains the only definitive therapy for patients unable to regenerate sufficient hepatocyte mass in a timely manner. The advent of OLT has coincided with a rise in overall ALF survival rates from approximately 20% in the pretransplantation era to >70% at the present time. One-year posttransplantation survival for patients with ALF has been reported to be as high as 80% to 90%.21 Although these improvements in survival rates are impressive, it should be noted that 10% of patients still die while awaiting OLT, which confirms that the potential for improved patient outcome still has not been realized because of the ongoing liver allograft shortage.
As mentioned earlier, patient survival could be improved if additional time could be gained for the patient while awaiting liver replacement or hepatocyte regeneration. The development of a support device to replace the acutely failing liver has been a highly sought after (and elusive) goal. Several systems have been tested without definitive evidence of efficacy. Transient improvement in hepatic encephalopathy has been observed in several trials, but improvement in hepatocyte function and long-term benefit have not been realized.31 Liver support trials are difficult to perform due to access to liver replacement, the rarity of affected patients, and the heterogeneous causes and varying levels of disease severity. Therefore, additional data are necessary, and liver support systems should be used only as part of an approved clinical trial. Focus has now shifted toward xenotransplantation,32 organ engineering, and cell transplantation.33 In the meantime, living donation and auxiliary liver transplantation can help overcome the organ shortage.34
CIRRHOSIS AND PORTAL HYPERTENSION
Cirrhosis, the final sequela of chronic hepatic insult, is characterized by the presence of fibrous septa throughout the liver subdividing the parenchyma into hepatocellular nodules (Fig. 31-13).35 Cirrhosis is the consequence of sustained wound healing in response to chronic liver injury. Approximately 40% of cirrhotic patients are asymptomatic, but progressive deterioration leading to the need for liver transplantation or death is typical after the development of end-stage liver disease (ESLD). The complications of ESLD include progressive hyperbilirubinemia, malnutrition, decreased synthetic function of the liver, coagulopathy, portal hypertension (i.e., ascites and variceal bleeding), hepatic encephalopathy, and life-limiting fatigue. ESLD carries a 5-year mortality of 50%, with 70% of deaths due to liver failure.36 In the United States, cirrhosis accounts for 30,000 deaths per year and is the most common nonneoplastic cause of death among patients with hepatobiliary and digestive diseases. An additional 10,000 to 12,000 deaths occur annually due to HCC, the most rapidly increasing neoplasm in the United States.36.
Morphologically, cirrhosis can be described as micronodular, macronodular, or mixed. Micronodular cirrhosis is characterized by thick regular septa, small uniform regenerative nodules, and involvement of virtually every hepatic lobule. Macronodular cirrhosis frequently has septa and regenerative nodules of varying sizes. The regenerative nodules consist of irregularly sized hepatocytes with large nuclei and cell plates of varying thickness. Mixed cirrhosis is present when regeneration is occurring in a micronodular liver and over time converts to a macronodular pattern. This morphologic categorization is limited, and cirrhosis is a dynamic process in which nodule size varies over time. The three patterns correlate poorly with etiology, and the same pattern can result from a variety of disease processes. Conversely, a single disease process can demonstrate several morphologic patterns. Irrespective of etiology and morphologic pattern, the cirrhotic liver frequently demonstrates right hepatic lobe atrophy, caudate lobe and left lateral segment hypertrophy, recanalization of the umbilical vein, a nodular surface contour, dilatation of the portal vein, gastroesophageal varices, and splenomegaly on radiographic evaluation.
Cirrhosis can result from a wide range of disease processes, including viral, autoimmune, drug-induced, cholestatic, and metabolic diseases (Table 31-3). In the diagnosis of alcoholic liver disease, documentation of chronic alcohol abuse is imperative. Liver biopsy will reveal the typical findings of alcoholic hepatitis, including hepatocyte necrosis, Mallory bodies, neutrophil infiltration, and perivenular inflammation. Patients with nonalcoholic steatohepatitis (NASH) often endorse a history of diabetes mellitus or metabolic syndrome. The diagnosis of NASH requires the demonstration of steatohepatitis on biopsy, the lack of a history of significant alcohol consumption, and exclusion of other causes of hepatic steatosis. Although cryptogenic cirrhosis, or cirrhosis without an apparent cause, accounted for a third of all cases in the past, this proportion has declined over time as it becomes increasingly apparent that many of such patients may actually have unrecognized NASH.
Viral hepatitis (hepatitis B, C, and D) Cryptogenic Alcohol abuse Metabolic abnormalities Iron overload (hemochromatosis) Copper overload (Wilson’s disease) α1-Antitrypsin deficiency Glycogen storage disease (types IA, III, and IV) Tyrosinemia Galactosemia Cholestatic liver disease Hepatic vein outflow abnormalities Budd-Chiari syndrome Cardiac failure Autoimmune hepatitis Toxins and drugs |
Chronic hepatitis C infection is the most common cause of chronic liver disease and the most frequent indication for liver transplantation in the United States. The identification of chronic hepatitis C infection is facilitated by serologic assays that detect antibody to hepatitis C and molecular assays that quantify hepatitis C viral RNA. Chronic hepatitis B, on the other hand, can be diagnosed based on the detection of hepatitis B surface antigen (HBsAg) more than 4 to 6 months after initial infection. Additional tests of hepatitis B viral replication, such as the hepatitis B e antigen (HBeAg) and hepatitis B viral DNA, can be used to confirm ongoing infection and to guide appropriate antiviral therapy.
Autoimmune causes of cirrhosis include primary biliary cirrhosis, primary sclerosing cholangitis, and autoimmune hepatitis. Patients with primary biliary cirrhosis may be asymptomatic or may present with a history of fatigue, pruritus, and skin hyperpigmentation that is not related to jaundice. Antimitochondrial antibodies will test positive in the vast majority of cases. Affected patients also may have marked elevations in serum cholesterol, while hyperbilirubinemia is seen late in the course of the disease. Primary sclerosing cholangitis is a chronic cholestatic disease of the liver associated with ulcerative colitis and Crohn’s disease. The clinical presentation can include pruritus, steatorrhea, fat-soluble vitamin deficiencies, and metabolic bone disease. The diagnosis is often established by imaging of the biliary tree, which reveals a characteristic picture of diffuse, multifocal strictures with focal dilation of the bile ducts resulting in a beaded appearance. Complications are common and can include biliary strictures, cholangitis, cholelithiasis, and cholangiocarcinoma. Autoimmune hepatitis is often accompanied by an elevation in serum globulins, particularly gamma globulins. Liver biopsy will show nonspecific changes such as a portal mononuclear cell infiltrate with the characteristic presence of plasma cells. Many patients with autoimmune hepatitis will respond to treatment with prednisone with or without azathioprine.
Hereditary hemochromatosis is the most common metabolic disorder causing cirrhosis. This entity should be suspected if the patient’s clinical presentation includes skin hyperpigmentation, diabetes mellitus, pseudogout, cardiomyopathy, or a family history of cirrhosis. Elevated plasma ferritin and increased iron saturation levels suggest the presence of iron overload, but these findings also can be seen in other diseases of the liver. Confirmatory testing can be achieved by means of genetic testing, liver biopsy, or by assessing the response to phlebotomy. Other uncommon metabolic disorders leading to cirrhosis include Wilson’s disease and α1-antitrypsin deficiency.
The clinical history associated with cirrhosis can include fatigue, anorexia, weight loss, jaundice, abdominal pain, peripheral edema, ascites, GI bleeding, and hepatic encephalopathy. On physical examination, a number of findings have been described in patients with cirrhosis. Spider angiomata and palmar erythema are believed to be caused by alterations in sex hormone metabolism. Finger clubbing may be a consequence of hypoalbuminemia, while the pathogenesis of white nail beds and Dupuytren’s contractures are less well understood. Males may develop features of feminization such as gynecomastia, loss of chest and axillary hair, and testicular atrophy. Splenomegaly is common, whereas the cirrhotic liver itself may be enlarged, normal sized, or small. Ascites and pleural effusion can be seen with fluid accumulation. Portal hypertension can manifest as caput medusae and/or the presence of the Cruveilhier-Baumgarten murmur, a venous hum that can be auscultated in the epigastrium resulting from collaterals between the portal system and the remnant of the umbilical vein. Jaundice usually does not appear until the bilirubin rises above 2 to 3 mg/dL. Asterixis can be detected in patients with hepatic encephalopathy. Other manifestations include fetor hepaticus, as well as features suggestive of malnutrition such as weakness, weight loss, and temporal muscle wasting.
Although fat stores and muscle mass are reduced, resting energy expenditure is increased. Muscle cramps occur frequently in the cirrhotic patient and are felt to correlate with ascites, low mean arterial pressure, and plasma renin activity. Abdominal hernias are common with ascites and should be electively repaired only in patients with well-compensated cirrhosis; otherwise, the hernia should be repaired at the time of or after hepatic transplantation. HCC can occur in all forms of cirrhosis, and every cirrhotic patient should undergo screening for the development of HCC every 6 months via imaging and measurement of a serum α-fetoprotein (AFP) level. Cirrhosis is associated with increased cardiac output and heart rate as well as decreased systemic vascular resistance and blood pressure. Patients with cirrhosis are more prone to infections due to impaired phagocytic activity of the reticuloendothelial system. Bacterial infections, often of intestinal origin, are common and must be suspected in a patient with unexplained pyrexia or clinical deterioration. Spontaneous bacterial peritonitis also is seen in cases of cirrhosis with ascites. Intrinsic drug metabolism is reduced in the cirrhotic liver, and this fact needs to be recognized when prescribing medications.
Laboratory findings vary in the cirrhotic patient depending on the degree of compensation; however, in general, a number of trends are seen. The cirrhotic patient usually has a mild normocytic normochromic anemia. The white blood cell and platelet counts are reduced, and the bone marrow is macronormoblastic. The PT is prolonged and does not respond to vitamin K therapy, and the serum albumin level is depressed. Urobilinogen is present and urinary sodium excretion is diminished in the presence of ascites. The serum levels of bilirubin, transaminases, and alkaline phosphatase may all be elevated. However, normal liver function test results do not eliminate the possibility of cirrhosis.
The diagnosis of cirrhosis can be made in many cases from a constellation of clinical features, laboratory values, and radiographic findings. Histopathologic examination of liver tissue is occasionally needed to confirm the diagnosis of cirrhosis and in determining disease etiology, activity, and progression. Liver biopsy can be performed via a percutaneous, transjugular, or laparoscopic approach. If needed, ultrasound or CT guidance can be helpful in obtaining an adequate sample and avoiding other viscera.
Various serologic markers of hepatic fibrosis are currently being investigated to help predict the presence of cirrhosis without the need for liver biopsy. However, no currently available marker is sufficiently accurate for clinical use. Ultrasound elastography, which measures the stiffness of the liver by inducing an elastic shear wave that propagates through the tissue, shows promise as a noninvasive test in identifying patients with advanced fibrosis and cirrhosis. (See earlier section, “Radiologic Evaluation of the Liver.”)
Assessing the hepatic reserve of the cirrhotic patient is important, because cirrhosis and portal hypertension can have a negative impact on the outcome of nontransplant surgical procedures. Patients with liver disease undergoing surgery are at increased risk for surgical and anesthesia-related complications. The actual risk depends on the type of anesthetic used, the specific surgical procedure performed, and the severity of liver disease. Previous studies have demonstrated that emergency operations, cardiac surgery, hepatic resections, and abdominal surgery, particularly cholecystectomy, gastric resection, and colectomy, generate the highest operative risk among cirrhotic patients. Additionally, preoperative patient characteristics such as anemia, ascites, encephalopathy, malnutrition, hypoalbuminemia, hypoxemia, infection, jaundice, portal hypertension, and prolonged PT have also been associated with inferior outcomes after surgery. Nontransplant surgical procedures are contraindicated in patients with acute fulminant hepatitis and those with severe decompensated chronic hepatitis.
A number of laboratory tests have been used to assess hepatic reserve in patients with cirrhosis. Tests of indocyanine green, sorbitol, and galactose elimination capacity as well as the carbon-13 galactose breath test and carbon-13 aminopyrine breath test have all been disappointing clinically due to their dependence on flow to the liver as well as the unavailability and complexity of the tests. The monoethylglycinexylidide (MEGX) test, which measures MEGX formation after the administration of lidocaine, has been shown to be approximately 80% sensitive and specific in diagnosing cirrhosis. However, this test loses both sensitivity and specificity as the serum bilirubin level rises and interferes with the fluorescent readout system.
The Child-Turcotte-Pugh (CTP) score was originally developed to evaluate the risk of portocaval shunt procedures performed for portal hypertension and subsequently has been shown to be useful in predicting surgical risks of other intra-abdominal operations on cirrhotic patients (Table 31-4). Numerous studies have demonstrated overall surgical mortality rates of 10% for patients with class A cirrhosis, 30% for those with class B cirrhosis, and 75% to 80% for those with class C cirrhosis.37 The CTP score is derived from five variables as shown in Table 31-4. The problems with the CTP score are the presence of subjective variables (encephalopathy and ascites), its narrow range (5 to 15 points), and the equal weighting given to each variable. Multiple retrospective studies have demonstrated that perioperative mortality and morbidity rates correlate well with the CTP score, and for over 30 years, this measure had been used as the principal predictor of operative risk.
VARIABLE | 1 POINT | 2 POINTS | 3 POINTS |
---|---|---|---|
Bilirubin level | <2 mg/dL | 2–3 mg/dL | >3 mg/dL |
Albumin level | >3.5 g/dL | 2.8–3.5 g/dL | <2.8 g/dL |
International normalized ratio | <1.7 | 1.7–2.2 | >2.2 |
Encephalopathy | None | Controlled | Uncontrolled |
Ascites | None | Controlled | Uncontrolled |
Child-Turcotte-Pugh class | |||
Class A = 5–6 points | |||
Class B = 7–9 points | |||
Class C = 10–15 points |
The Model for End-Stage Liver Disease (MELD) is a linear regression model based on objective laboratory values (INR, bilirubin level, and creatinine level). It was originally developed as a tool to predict mortality after transjugular intrahepatic portosystemic shunt (TIPS) but has been validated and used as the sole method of liver transplant allocation in the United States since 2002. The MELD formula is as follows:
where Ln represents natural logarithm, SCr is serum creatinine level (in milligrams per deciliter), and Tbil is serum bilirubin level (in milligrams per deciliter).
A number of studies have examined the relative values of MELD and CTP scores in predicting postoperative mortality in cirrhotic patients undergoing nontransplant surgical procedures. Northup and colleagues demonstrated that MELD score was the only statistically significant predictor of 30-day mortality.38 In this study, mortality increased by approximately 1% for each MELD point up to a score of 20 and by 2% for each MELD point above 20. A comparison of the MELD model with the CTP classification showed good correlation between the two measures in predicting mortality, especially in the setting of emergency surgery.39 In these studies, the relative risk of mortality increased by 14% for each 1-point increase in MELD score. As a result, it has been proposed that patients with a MELD score below 10 can safely undergo elective surgery, those with MELD between 10 and 15 may undergo surgery with caution, while those with MELD scores in excess of 15 should not be subjected to elective surgical procedures.40
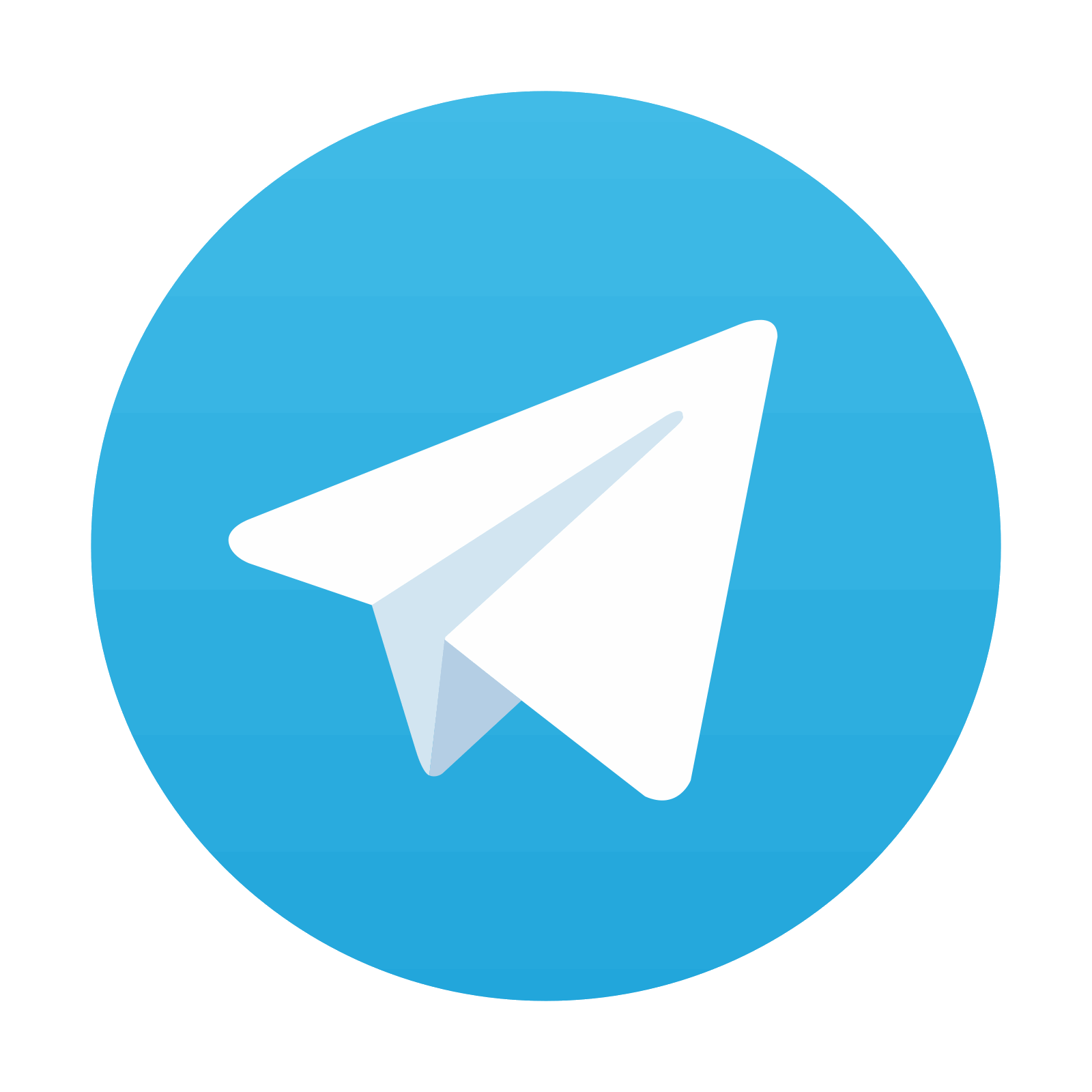
Stay updated, free articles. Join our Telegram channel
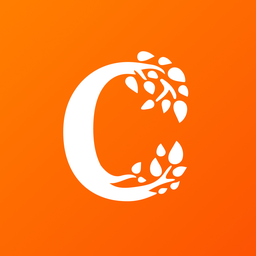
Full access? Get Clinical Tree
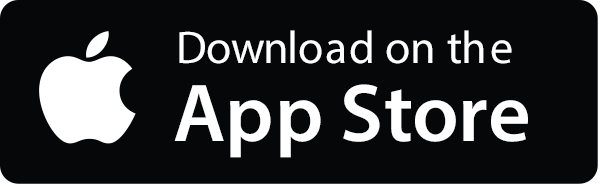
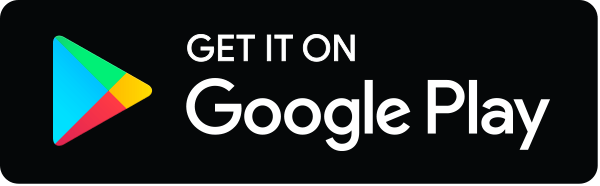