.c-ckc-abstract{border-bottom:1px solid #d7d7d7;margin-bottom:2em;padding-bottom:1em}.c-ckc-acknowledgment{background-color:#e7e7e7;margin-top:2em;padding:1em}.c-ckc-appendices{border-top:1px solid #d7d7d7;margin-top:2em;padding-top:2em}.c-ckc-article-footnote{margin-top:1em}.c-ckc-author-group{font-weight:700}.c-ckc-bibliography__header{margin-top:1.5em}.c-ckc-view-in-source-link{margin-right:1em}.c-ckc-def-list-item{border-bottom:1px dotted #d7d7d7;margin-bottom:1.5em;padding-bottom:.5em}.c-ckc-figure{margin:1.5em 0}.c-ckc-figure__image{height:auto;max-width:100%}.c-ckc-figure__caption,.c-ckc-figure__label,.c-ckc-figure__source{color:#737373;font-size:.875em;margin-bottom:.5em}.c-ckc-figure__label{font-weight:700;text-transform:uppercase}.c-ckc-footnote{color:#737373;font-size:.875em;margin:.5em 0}.c-ckc-formula{margin:1em;overflow-x:auto;overflow-y:hidden;text-align:center}.c-ckc-further-reading__header{margin-top:1.5em}.c-ckc-journal-head-matter{border-top:1px solid #d7d7d7;margin-top:2em;padding-top:2em}.c-ckc-list{padding-left:1em}.c-ckc-list__item{list-style-type:none}.c-ckc-list__item .c-ckc-list{margin-top:1em}.c-ckc-list__item-label{float:left;margin-right:.5em}.c-ckc-inline-reference+.c-ckc-inline-reference{margin-left:.25em}.c-ckc-section__label{float:left;margin:0 .5em 0 0}.c-ckc-table{margin:1.5em 0}.c-ckc-table__table{border:1px solid #dcdcdc;border-collapse:collapse}.c-ckc-table__caption,.c-ckc-table__footnote,.c-ckc-table__label,.c-ckc-table__legend{color:#737373;font-size:.875em;margin-bottom:.5em}.c-ckc-table__label{font-weight:700;text-transform:uppercase}.c-ckc-table__superscript-label{color:#007398}.c-ckc-table__overflow{margin:.5em 0;overflow:auto}.c-ckc-table__header-cell{background-color:#ebebeb;font-weight:700;border:1px solid #dcdcdc;padding:.25em;text-align:left;vertical-align:top}.c-ckc-table__body-cell{background-color:#fff;border:1px solid #dcdcdc;padding:.25em;vertical-align:top}.c-ckc-textbox{background-color:#dff8ff;margin:1.5em 0}.c-ckc-textbox__caption,.c-ckc-textbox__label,.c-ckc-textbox__legend,.c-ckc-textbox__source,.c-ckc-textbox__subtitle,.c-ckc-textbox__title{color:#737373;font-size:.875em;margin-bottom:.5em}.c-ckc-textbox__label{font-weight:700;text-transform:uppercase}.c-ckc-textbox__body{padding:1em 1.5em}.c-ckc-textbox__tail{margin-top:1em}.u-ckc-small-cap{font-variant:small-caps}.u-ckc-monospace{font-family:monospace}.u-ckc-superscript{line-height:1}.u-ckc-unstyled-list{list-style-type:none}
Infections are one of the most frequent serious complications of hematopoietic stem cell transplantation (HSCT). The risk of infections corresponds to the complex interplay between organ dysfunction or tissue damage, exposure to pathogens, virulence of those pathogens, and the net state of immunosuppression. Although all of these factors are interrelated and each contributes to infection risk in some way, the time to recovery of the immune system is the most significant determinant of infection risk. The recovery of different components of the immune system is variable; therefore infection risk is often considered in time periods relative to transplantation. Traditionally, these periods have been considered fixed and are categorized as pre-engraftment (days 0 to 30), early post-engraftment (days 30 to 100), and late post-engraftment (days 100+) time periods ( Fig. 2.1 ). Anchoring infection risk to these time periods can help establish a general construct for infection risk for bacteria, viruses, and fungi across the time periods. However, it is important that clinicians recognize that the timing of recovery for specific components of the immune system can vary considerably from one patient to another and thus fixed risk periods may not be ideal. For example, the duration of neutropenia corresponds with an increased risk of invasive fungal disease and is associated with the patient’s indication for transplantation, extensiveness of prior therapy, stem cell source, cell dose, conditioning regimen, and graft failure or rejection if it occurs. It is more difficult to establish patient-specific or disease-specific immune recovery time periods; however, this knowledge will help guide the clinician through a more nuanced clinical assessment for a patient at a specific point in time after transplantation. This chapter aims to provide the clinician with the ability to assess infection risk using both the fixed time period approach as well as an individualized patient specific approach.

Infection risk by fixed time periods after transplantation
Pre-engraftment period
The pre-engraftment period is often considered to correspond to days 0 to 30 after HSCT; however, this period can include days before neutrophil engraftment as well as days soon after. The infection risk in this early period is attributed to many factors, including neutropenia, the breakdown of mucosal barriers leading to subsequent microbial invasion, and acute graft-versus-host disease (GVHD) leading to further barrier breakdown and immunosuppression. Patients receiving myeloablative conditioning are most vulnerable in this early period owing to longer durations of neutropenia and mucosal barrier injury associated with myeloablative regimens. The majority of infections are monomicrobial and are most often the result of bacterial pathogens. The most common isolated organisms include coagulase-negative staphylococci, Enterococcus species, Staphylococcus aureus , or enteric gram-negative bacilli. The portal of entry for many of these organisms is either via a central venous catheter or translocation of a compromised mucosal barrier, often secondary to myeloablative conditioning regimens. Oral mucosal barrier injury can predispose specifically to viridans group streptococci; however, mucosal barrier injury can include any part of the alimentary tract from the oral cavity to the anus. Clostridium difficile infection is one of the most common causes of infectious diarrhea after HSCT given frequent exposure to precipitating factors such as chemotherapy and antibiotics.
Invasive fungal disease, either yeast or molds, tends to occur in a biphasic pattern after HSCT with the first risk period presenting in this pre-engraftment phase. The most common fungal pathogens are Candida or Aspergillus species. More recent use of supportive care such as antifungal prophylaxis has led to a reduction of these events in the pre-engraftment period; however, breakthrough invasive fungal disease from genera other than Candida or Aspergillus may occur and, more rarely, resistance may occur.
The next most frequently involved pathogens are viruses, which commonly include cytomegalovirus (CMV), herpesviruses, adenovirus, BK virus, respiratory viruses, and gastrointestinal viruses. Antiviral prophylaxis for some of these viral infections may delay or postpone the presentation into the early post-engraftment or late post-engraftment periods. CMV is the most common viral infection encountered in the posttransplant period. Antiviral prophylaxis has limited utility in the immediate posttransplant period owing to associated toxicities with current options. This differs from varicella zoster virus and herpes simplex virus, members of the herpesvirus family frequently encountered after transplant, for which institutions often use antiviral prophylaxis with acyclovir for the first year to prevent reactivation. , Other herpesvirus infections (Epstein-Barr virus, human herpes virus type 6, and, less commonly, human herpes virus type 7 and human herpes virus type 8) can also be identified in this period but far less frequently. Although adenovirus and BK virus are not members of the herpesvirus family, these viruses can maintain a persistent asymptomatic state before transplantation and reactivation can occur in the early and late posttransplant periods. Lastly, hospital- and community-acquired respiratory (i.e., respiratory syncytial virus, rhinovirus, influenza) and gastrointestinal (i.e., norovirus, astrovirus) viral infections can be encountered in this early posttransplant period, most without any adequate treatment or prophylaxis, and can be devastating.
Early post-engraftment period
In the early post-engraftment period, neutropenia has resolved, which is an important milestone for reduced vulnerability to bacterial and fungal pathogens. However, the clinician should still be alert to opportunistic infections from these pathogens owing to risk factors, such as continued need for central venous access, residual mucositis, intermittent neutropenia secondary to medication toxicity, or more rarely, graft loss. Persistent lymphopenia and slow T-cell reconstitution is primarily responsible for vulnerability to infections during this period. Poor T-cell reconstitution can be further delayed by the need for immune suppressive agents to manage HSCT complications such as acute GVHD. This combination of poor T-cell function and need for additional immune suppression predisposes children to an increased risk of latent viral reactivations, poor outcomes from typically self-limiting primary viral infections, and invasive mold disease. Additionally, this period is an important window of risk for Pneumocystis jirovecii pneumonia; therefore all patients continue prophylaxis through this period and often until T-cell reconstitution.
Late post-engraftment period
The late post-engraftment period starts 100 days after HSCT but varies in duration owing to the individual patient requirement for ongoing immunosuppression and delayed immune reconstitution. Patients may remain at high risk for infections because of prolonged immunosuppression secondary to treatment for chronic GVHD or autoimmune cytopenias. This results in delayed reconstitution of both cellular and humoral immunity. Bacteremia, sinusitis, upper respiratory tract infections, pneumonia, and meningitis are not infrequently caused by encapsulated bacteria ( Streptococcus pneumoniae , Haemophilus influenzae , Neisseria meningitidis ) during this period. Patients with chronic GVHD are particularly susceptible to these infections owing to poor opsonophagocytosis and hyposplenism, referred to as functional asplenia. Therefore some institutions initiate antibiotic prophylaxis for functional asplenia to prevent overwhelming bacterial sepsis. In addition to encapsulated organisms, bacteremia during this period may also result from Staphylococcus species or gram-negative bacteria. Factors that may predispose patients to bacteremia from these pathogens include the continued presence of central venous access or persistent mucosal barrier dysfunction. Although the peak of reactivation of latent viruses is in the early post-engraftment period, the risk persists through this late phase. For Epstein-Barr virus, reactivation can lead to the development of posttransplant lymphoproliferative disease, which typically presents between 3 and 5 months after transplant. Other atypical late post-engraftment infections may be due to Nocardia species, Listeria species, Cryptococcus species, and nontuberculous mycobacteria. Finally, the risk for P. jirovecii pneumonia can remain well after 100 days from HSCT, particularly if continued immune suppression is required.
Approaches to prophylaxis relative to infectious risk periods
In general, there are three methods to provide posttransplant antimicrobial prophylaxis: pharmacologic prophylaxis, immunoprophylaxis (immunoglobulin [IG] replacement therapy), and vaccinations. For each method, there are two approaches to guide initiation or duration of prophylaxis: a uniform time at risk approach or an individualized approach that takes into account ongoing immunosuppression and immune reconstitution. With the uniform time-at-risk approach, antimicrobial prophylaxis is continued until a designated time period elapses. An example would be the use of antifungal prophylaxis until day 100 (which may be extended for patients requiring immunosuppressive treatment for GVHD). Much of the data on the effectiveness for prophylaxis have evolved from trials designed with this simpler standardized time-at-risk approach. Although it has the advantage of consistency and ease of use, this approach likely results in overtreatment of some patients and undertreatment of others.
The second approach is individualization of the duration of time during which prophylaxis is provided or of the timing at which vaccinations are administered based on an assessment of an individual patient’s cellular and humoral immunity. This system is much more cumbersome and has less evidence to support its use; however, it should theoretically result in earlier discontinuation of prophylaxis for some patients with adequate immune reconstitution and appropriately prolong prophylaxis for patients with immune defects that persist beyond an estimated risk period duration from transplantation. Examples of this approach include continuation of antifungal or antiviral prophylaxis until patients achieve functional cluster of differentiation (CD)4 + T-cell reconstitution or discontinuation of IG replacement therapy when patients have adequate CD19 + B cells, switched memory B cells, and evidence of IgM and IgA production. Whether a center uses the fixed time-at-risk approach or a more individualized approach will depend on the infrastructure of the transplant center and its ability to consistently apply a more nuanced approach to prophylaxis.
After HSCT and Ig replacement therapy is discontinued, recipients must be revaccinated. There are limited data on vaccine efficacy and ideal timing of vaccinations in HSCT recipients; however, it is accepted that there must be at least partial recovery of T and B cells before administration. Vaccination with polysaccharide antigen vaccines elicits T-cell–independent antibody responses and therefore typically fails to produce protective immunity in most allogeneic HSCT recipients within the first year after transplantation. However, conjugate vaccines evoke T-cell–dependent antibody responses and produce protective antibody responses within the first year after allogeneic HSCT even with patients receiving immunosuppression. Therefore most revaccination guidelines are based on timing from transplantation, and HSCT recipients could undergo early revaccination with conjugate vaccines analogous to newborn vaccination schedules and achieve protective long term immunity. Vaccination with inactivated or toxoid-containing vaccines is recommended as early as 3 to 6 months after HSCT, whereas administration of live-attenuated vaccines is recommended at 24 months after HSCT. The delayed use of live-attenuated vaccines is based on concerns about transmission of vaccine-mediated disease and limited clinical data on safety or immunogenicity of earlier vaccination. The Advisory Committee on Immunization Practices to the Center for Disease Control and Prevention and the Infectious Diseases Society of America publish time-based guidelines on vaccination after transplantation, but vaccine schedules vary greatly among institutions.
Timing of immune reconstitution after hematopoietic stem cell transplantation
Immune reconstitution after HSCT involves the recovery of both hematopoietic and immunologic function. This occurs in several phases, resulting in recovery of specific components of the immune system at distinct time points ( Table 2.1 ).
Auto-PBSC | Allo-PBSC | Allo-BM | Allo-UCB | |
---|---|---|---|---|
Ease of collection | Recipient requires stem cell mobilization and central line apheresis | Donor requires stem cell mobilization and central line apheresis | Donor anesthesia | Very safe |
Time to neutrophil engraftment (ANC >500) | Very fast (7-14 days) | Fast (14-21 days) | Slow (17-24 days) | Very slow (24-42 days) |
T-cell reconstitution a | 30 days | 6-12 months | 3-12 months | 3-12 months |
B-cell reconstitution b | 60 days | 4-12 months | 3-12 months | 2-6 months |
Graft versus host disease | None | Common in mismatched grafts; increased risk of chronic GVHD compared with blood marrow | Less common | Uncommon or mild |
HLA matching | N/A | Requires T-cell depletion for HLA mismatch | Requires HLA-identical match c | HLA mismatch well tolerated |
HSC numbers | High | High | High (depending on host-donor weights) | Low |
a T-cell reconstitution defined as CD4 count >200/μL. T-cell reconstitution is highly variable and dependent on T-cell depletion, HLA match, and the development of acute graft-versus-host disease.
b B-cell reconstitution defined as >200/μL.
c Mismatched blood marrow HSCT can be performed but less optimal.
Innate immune recovery after transplantation
The innate immune system can be divided into nonhematopoietic and hematopoietic compartments. The nonhematopoietic compartment includes physical barriers, such as the skin and mucosal surfaces, which can be damaged during transplantation by the pretransplant conditioning regimen. However, the damage is typically restored soon after transplantation. Repair can be inhibited by GVHD of the skin or mucous membranes. The skin and mucosal barriers can also be compromised by the presence of foreign material, such as a central venous catheter or a gastrostomy tube, for prolonged periods after transplantation.
Hematopoietic innate immune cells include neutrophils, macrophages, as well as natural killer (NK) cells. After myeloablative conditioning, patients undergo an aplastic phase, which is identified by severe neutropenia, anemia, and thrombocytopenia. The first laboratory sign of hematologic recovery is typically neutrophil recovery. Engraftment, classically defined as absolute neutrophil count greater than 500/μL, is typically achieved between 10 and 42 days and transplant, depending on the stem cell source (see Table 2.1 ). Hematopoietic growth factors, such as granulocyte colony-stimulating factor (G-CSF), can be used to accelerate recovery of granulocyte counts, minimize the duration of neutropenia, and decrease the risk for severe infections. The use of G-CSF after HCT is universal in the autologous setting but is more controversial in allogeneic graft recipients owing to a lack of benefit in reducing mortality. Most centers use G-CSF for recipients of umbilical cord blood (UCB) transplants, whereas its use in others is more variable as there are concerns that G-CSF may increase rates of GVHD or malignant relapse. However, administration of certain post-HSCT medications (such as ganciclovir or valganciclovir) may result in secondary neutropenia. Furthermore, the neutrophils may have abnormal function for up to 2 months after transplant.
Monocytes are leukocytes that circulate peripherally until they eventually migrate into tissues where they develop into macrophages and dendritic cells. Monocytes, macrophages, and dendritic cells function through phagocytosis, a process that is particularly important for pathogen killing and tissue repair. However, mononuclear phagocytes also activate the adaptive immune system via antigen presentation and cytokine production. Posttransplant neutrophil recovery is occasionally preceded by the detection of peripheral monocytes; however, monocyte function may remain suboptimal for up to 1 year after transplant. Although monocyte function is difficult to measure in clinical laboratories, based on data extrapolated from animal models, it is thought that tissue macrophages and dendritic cells are not significantly depleted as a result of transplant conditioning, and natural turnover results in their being gradually replaced by donor-derived cells for up to a year after HSCT. Peripheral dendritic cells can also be detected at the time of neutrophil count recovery and a low dendritic cell count at engraftment may predict relapse, death, and acute GVHD.
NK cells are also key members of the innate immune system that influence adaptive function. Derived from the lymphoid lineage, NK cells have a unique function in the prevention of viral infections and antitumor immunity. NK cell reconstitution varies by graft source. NK cells recover in number and function in the first few weeks after allogeneic HSCT, much earlier than T- and B-cell reconstitution, which is likely related to the high IL-2 levels during the early posttransplant period. Not only do NK cells appear early, they also acquire functional competence much earlier than other lymphocytes. Furthermore, NK cell activity after HSCT remains normal even in the presence of severe GVHD.
Delay in neutrophil engraftment greatly increases morbidity, and failure of sustained neutrophil engraftment after a myeloablative-conditioning regimen requires urgent retransplantation. Engraftment failure can occur from inadequate hematopoietic stem cell quantity from poor collection or loss in postcollection processing, inadequate host support of infused cells, posttransplantation events such as infection or medications, or from host-versus-graft (immunologic) rejection. Engraftment failure is a very rare complication of autologous transplantation and is likely only present in the setting of poor cryopreservation of stem cells. For allogeneic HSCT, the risk of engraftment failure is dependent on a number of variables, including baseline host immunity, HLA disparity, type of conditioning regimen and stem cell source used, low stem cell dose, ex vivo T-cell depletion (TCD), ABO incompatibility, and disease status at transplantation. Patients with hematologic malignancies have a rejection risk of approximately 5%, whereas the risk may be greater than 10% in patients with nonmalignant conditions. Graft rejection is more common in haploidentical related and mismatched unrelated donor (URD) transplants and much less frequent in matched sibling donor transplantation. Generally, UCB transplant recipients have the highest risk of graft failure, whereas peripheral blood stem cell grafts have the lowest risk of graft rejection. The incidence of graft failure also varies considerably among institutions owing to differing approaches to conditioning.
Adaptive cellular immune recovery after transplantation
Often clinicians are reassured about a patient’s infection risk once neutrophil engraftment is achieved. Although neutrophil recovery is an important milestone, patients continue to be vulnerable to opportunistic infection because of persistent cellular immunodeficiencies involving the adaptive immune system. The recovery of the adaptive immune system is much more nuanced, involving refinement and adjustment of T and B lymphocytes over the lifetime of an individual. After HSCT, T and B lymphocytes reconstitute slowly and develop both a cellular and humoral response. The cellular immune response to pathogens is initiated by antigen-presenting cells (e.g., macrophages and dendritic cells) but also requires the presence of functional T cells for activation. HSCT results in impairment of the adaptive immune response through loss of naïve T cells and reduced function of existing T cells. The recovery of the T-cell compartment initially relies on peripheral expansion of infused donor memory T cells, which leads to a narrow T-cell receptor repertoire. This process is driven by cytokines, such as IL-7 and IL-15, as well as by antigen stimulation and T-cell receptor (TCR) engagement. Peripheral T-cell expansion is eventually followed by the production of naïve T cells in the thymus leading to a population of memory T cells with a diverse TCR repertoire. In patients receiving T-cell–replete grafts, peripheral expansion of infused memory T cells with a limited repertoire occurs initially until hematopoietic progenitors seed the thymus and produce T cells with a more diverse repertoire. In T-cell–depleted transplants, seeding of the thymus by hematopoietic progenitors is the primary route to T-cell reconstitution.
In either route, effective long-term and sustained T-cell lymphopoiesis is dependent on the presence of a functional thymus. Thymic dependence in generating a diverse T-cell repertoire after HSCT is a critical hurdle in patients without a thymus or with a poorly functioning thymus. Aging, recurrent or chronic infections, chemotherapy, radiation exposure, and GVHD can all lead to thymic atrophy and subsequent difficulty with T-cell reconstitution. Detection of recent thymic immigrants and T-cell polyclonality are typical methods used to determine thymic function. TCR excision circles (TRECs) are small circularized portions of DNA created through T-cell maturation in the thymus, which can be used as a surrogate marker for reconstitution of thymus-derived CD4 + CD45RA + naïve T cells. However, these are just markers for thymic output and, in general, it is difficult to completely assess the function of the thymus after transplantation.
Naïve T-cell populations are usually reduced for long periods after HSCT. The inability to reconstitute the naïve T-cell compartment for several years after HSCT, in the absence of GVHD, is likely a consequence of both thymic dysfunction and impaired peripheral naïve T-cell homeostatic mechanisms and survival. CD4 + lymphocytes require a functional thymus for generation of CD4 + CD45RA + naïve T cells, whereas CD8 + lymphocytes are predominantly derived by clonal expansion outside the thymus. Therefore CD4 + T lymphocytes appear later than CD8 + T lymphocytes leading to the inversion of the CD4/CD8 ratio found after transplant. Inversion of the CD4/CD8 ratio is one of the earliest features of T-cell reconstitution after autologous or allogeneic transplantation from any graft source and can persist for up to several years after HSCT. . CD4 + T-cell reconstitution to a level of approximately 200/μL typically occurs around 3 months after HSCT but can vary considerably depending on the use of TCD methods, graft source (UCB), receipt of total body irradiation, or development of GVHD.
The development of regulatory T cells (Tregs) may be important in determining outcomes after allogeneic HSCT. Tregs suppress the activity of effector T cells, thus reducing inflammation and promoting immune homeostasis after allogeneic HSCT. The presence of donor Tregs enhances immune reconstitution and improves TCR diversity after transplantation. Increased donor Tregs are associated with a decreased risk of GVHD, and many studies have shown that Tregs are significantly reduced in HSCT recipients with GVHD. The relative predominance of effector T cells, compared with Tregs, leads to a proinflammatory milieu of cytokines. IL-6, characterized as both proinflammatory and antiinflammatory, is of particular interest in GVHD and moderates the differentiation of naïve T cells into either Tregs or effector T cells. IL-6 blockade promotes differentiation into Tregs and may mitigate the severity of GVHD. Tumor necrosis factor alpha (TNF-α) is typically classified as a proinflammatory cytokine; however, it may also have antiinflammatory properties mediated through its effects on Tregs. TNF-α has been shown to increase expansion, stability, and possibly the function of Tregs, and may therefore have conflicting effects on both GVHD incidence (high is bad early after HSCT) and severity (low is bad later after HSCT).
Adaptive humoral immune recovery after transplantation
The adaptive humoral immune response is mediated by antibodies and requires both functional T and B cells. In addition to the delayed recovery of T cells after HSCT, there is also impaired reconstitution of B cells. Impairment of B-lymphocyte number and function leads to absent Ig production and susceptibility to infections with encapsulated bacteria such as S. pneumoniae and H. influenzae . The B-cell compartment is the slowest to reconstitute. B-cell reconstitution depends on the intensity of conditioning. Typically, when myeloablative conditioning is administered, all B cells are genetically donor in origin but produced de novo from the bone marrow, and therefore do not retain immunologic memory from the donor. In reduced intensity conditioning, B cells may be of mixed host and donor origin, although data are lacking on whether the persistence of host B cells provides a bridge of immunologic memory. Typically, B-cell reconstitution occurs within 12 months but may take several years for complete development of memory B cells after allogeneic HSCT. Hematopoietic stem cells (HSCs) within the bone marrow undergo multiple stages of B-cell differentiation. Pro-B cells develop into pre-B cells and finally immature/transitional B cells. Transitional (CD19 + CD21 low CD38 high ) B cells are the first B cells emigrating from the bone marrow and are elevated in the peripheral blood in the first months after HSCT before progressively decreasing. Transitional B cells emigrate to the spleen where they differentiate into IgM + memory or mature B cells. Mature B cells migrate to the primary follicle of the lymph node and spleen for antigen exposure and differentiation into switched memory B cells or plasma cells. Reconstitution of switched memory B cells occurs upon antigen exposure from pathogens, the environment, or vaccines and requires CD4 + T-cell help for isotype switching. Therefore although naïve B cells reach normal levels by approximately 6 months after allogeneic HSCT, levels of IgM + memory B cells can remain low for up to 2 years. Much like the TCR repertoire, B-cell antibody diversity is severely diminished and suffers prolonged recovery, which is worsened by GVHD and by the medications used to treat it. Recovery of the B-cell count or specific antibody production is primarily of donor origin but can vary among types of allogeneic stem cell grafts, CD34 + cell doses, donor ages, or recipient ages.
After myeloablative conditioning, B cells are typically entirely of donor origin; however, plasma cells remain primarily of host origin in the first several months after transplant. Given the long-lived nature of plasma cells, it takes months to years to replace host plasma cells by newly produced donor plasma cells. Therefore institutions may consider continuation of Ig replacement therapy in HSCT recipients until there is adequate evidence of B-cell and plasma cell function as opposed to a predetermined period. Some considerations affecting discontinuation of Ig replacement may include absolute B-lymphocyte count, B-lymphocyte phenotyping, including percent of switched memory B cells (CD27 + IgM − IgD − ), IgM and IgA production, and isohemagglutinin production.
Autologous hsct as a model for immune reconstitution
Autologous hematopoietic stem cells can be given to rescue the bone marrow and immune system after high-dose chemotherapy toxicities, which can result in deep and prolonged bone marrow suppression. Infusion of autologous hematopoietic stem cells after high-dose chemotherapy can offer prolonged disease-free survival in hematologic malignancies, including Hodgkin and non-Hodgkin lymphomas, and distinct advanced pediatric tumors, such as brain tumors, neuroblastoma, and certain sarcomas. It requires the collection and storage of adequate HSC, preferably before alkylating agents or topoisomerase inhibitors. Immune reconstitution after allogeneic and autologous HSCT has some similarities; however, allogeneic HSCT carries a risk of graft failure as the result of immunologic rejection and involves a risk of GVHD, necessitating the use of immunosuppressive therapy to prevent and/or manage it. Autologous HSCT is therefore a model for immune reconstitution after transplantation because this method obviates known risk factors for impaired reconstitution, such as in vivo or ex vivo TCD, HLA disparity between donor and recipient, GVHD prophylaxis, occurrence of GVHD, and immunosuppressive therapy for GVHD.
Neutrophil engraftment occurs quickly after autologous transplantation, between 7 and 14 days (see Table 2.1 ). Although autologous HSCT recipients may have impaired thymic function owing to age-related involution, damage from chemotherapy, or injury from ionizing radiation, thymopoiesis is typically less affected, and there is a faster recovery of the naïve T-cell compartment compared with the allogeneic transplant recipients with similar conditioning regimens. There is faster recovery of CD3 + and CD4 + T cells as well as increased B- and NK-cell counts in the first posttransplant year. Normalization of T-cell number, lymphocyte proliferative responses to phytohemagglutinin, and IgM production occur in the majority of autologous HSCT recipients by 6 months after transplantation.
Earlier immune reconstitution corresponds to a decrease in severity and incidence of infections after autologous compared with allogeneic HSCT. The most common infections in the first year after transplantation include catheter-related bloodstream infection, varicella zoster virus infection, and pneumonia, but the majority of these infections occur in the first 6 months after autologous transplantation. In most children, supportive care measures, such as protective isolation and prophylactic antimicrobials, can be discontinued at 6 months after autologous transplantation as the risk of infection also decreases after that time.
Autologous transplantation of gene-modified hematopoietic stem cells, or gene therapy, is a novel approach to transplantation that involves the transfer of gene-corrected stem cells with ostensibly fewer immunologic complications and reduced toxicities from conditioning. Gene therapy is under investigation for a number of indications, including certain forms of severe combined immune deficiency in which patients lack the machinery necessary to produce lymphocytes. Patients who receive gene therapy for adenosine deaminase−deficient severe combined immune deficiency typically achieve immune reconstitution by 6 months after transplantation.
Factors affecting immune reconstitution, autoreactivity, and alloreactivity
The timing of immune reconstitution after HSCT, in particular after allogeneic HSCT, is affected by a number of variables, including HSCT procedure specific factors (e.g., HLA matching, source, conditioning regimen), pretransplant conditioning regimen, patient- and recipient-specific factors (e.g., age, sex, CMV status), and the presence and management of GVHD . The selection of a donor is a critical element contributing to the success of HSCT. There are several donor options, including identical twins (syngeneic, HLA-identical), the patient (autologous, HLA-identical), a sibling, relative URD (allogeneic HLA-matched, haploidentical, or mismatched), or UCB (allogeneic HLA matched or mismatched). Options for a donor depend on a number of variables with the goal of minimizing toxicities, decreasing risk of alloreactivity, and achieving adequate donor chimerism to lead to disease cure. These factors include the overall health and age of recipient and donor, disease progression, infection history, and clinical approach of the individual transplant center. There are many recipient and donor characteristics that could affect the timing of immune reconstitution and the subsequent infectious complications.
HLA matching
Donor and recipient matching are performed on human leukocyte major histocompatibility complex class I (HLA-A, -B, -C), and class II (HLA-DR, -DQ, -DP) antigens as a key part of successful allogeneic HSCT. These six loci contribute to graft-versus-host, graft-versus-tumor, and graft rejection responses. HLA-matched sibling donors are preferred for most transplants as they offer the best chance of engraftment and fastest immune reconstitution; however, there is only a 25% chance of having a matched sibling if siblings are present and available for donation. Other considerations for matched siblings include sibling age, source of collection (bone marrow or peripheral blood stem cells [PBSC]), and the possibility of the sibling donor being a carrier for the disease being treated. For example, carriers for the CYBB gene (X-linked chronic granulomatous disease) can have aberrations in neutrophil oxidative burst capacity and have been found to be at increased risk of infection or autoimmunity in adulthood and therefore should not be used as matched sibling donors. Similar considerations may exist for other X-linked diseases as well as for certain metabolic conditions, such as Hurler syndrome, in which carriers have only half the normal enzyme levels.
For patients without an HLA matched sibling, HSCT can be performed using a matched URD, a mismatched URD (single or double antigen), an UCB, or a haploidentical donor. The risks of acute and chronic GVHD and transplant-related mortality increase with the number of HLA mismatches, particularly in patients lacking HLA-A–, -B–, or -DRβ1–matched donors; however, HLA-C, -DQβ1, and -DPβ1 are also important. For transplant of nonmalignant diseases, URDs should ideally be matched at all 12 alleles. For transplant of malignant disease, fully matched URDs may have higher rates of relapse and decreased graft-versus-tumor effects; therefore some degree of mismatch may be beneficial. Disparity at HLA-A, -B, -C, and -DRβ1 alleles are definite risk factors for survival after URD transplantation, whereas single HLA-DQβ1 or -DPβ1 mismatches appear to be somewhat better tolerated. In addition to characterizing a suitable donor, homozygous HLA antigen mismatches can further be characterized as favoring either rejection (host versus graft or graft versus host). Furthermore, the use of partially HLA-mismatched allogeneic HSCs requires testing for circulating donor-specific HLA antibodies, as the presence of donor-specific antibodies increases the risk of primary graft failure and should be avoided whenever possible. If there is no alternative donor available, the recipient can potentially be desensitized to donor-specific antibodies before transplantation using plasmapheresis, Ig, and rituximab.
Although disease-free survival after URD transplantation continues to improve over the past decade, URD PBSC transplants are associated with delayed immune reconstitution and recipients are more likely to develop GVHD compared with recipients of matched sibling transplants, particularly if recipients lack an HLA-A–, -B–, or -DRβ1–matched donor. This has led to the use of more aggressive and prolonged immunosuppression for prophylaxis of GVHD for HSCT recipients of URD PBSC grafts, which further delays immune reconstitution. Transplantation from an URD has been associated with increased risk of predominantly late infections. By 1 year after HSCT, the number of recipients in whom at least one late infection developed, particularly infections from viruses, was increased compared with those who received a transplant from a matched related donor. This marked increase in late infections is the most important factor leading to increased nonrelapse mortality in URD transplantation.
Mismatched related (haploidentical) donors are often the only readily available donor for transplantation, which is essential in some diseases such as high-risk leukemias. However, recipients of unrelated or related mismatched donor HSCT have a higher rate of infectious complications than recipients of matched grafts, likely owing to the use of TCD methods. CMV and aspergillosis account for 40% of nonrelapse mortality in high-risk leukemia patients who receive haploidentical transplantation. More recent data show improved T-cell reconstitution with the use of posttransplant cyclophosphamide or the use of ex vivo combined CD3 + and CD19 + cell depletion in haploidentical HSCT, as these methods preferentially spare memory T cells. ,
Stem cell source
There are several potential sources for donor hematopoietic stem cells, which include bone marrow, peripheral blood stem cells, and cord blood stem cells, and the choice of stem cell source may affect immune reconstitution following HSCT.
Bone marrow.
For more than three decades, blood marrow has been the most frequent source of stem cells for transplantation. It is a safe procedure; however, for the donors, it is associated with considerable discomfort, fatigue, and longer recovery time than PBSC collections. The percentage of stem cells (CD34 + cells) among circulating total nucleated cells at steady state in bone marrow is approximately 18-fold higher than healthy donor peripheral blood. After bone marrow infusion, innate immunity usually recovers over the first several months. Reconstitution of adaptive immunity takes place over the first 1 to 2 years and is slower than PBSC transplantation.
Peripheral blood stem cells.
Mobilized PBSC have been widely used as a stem cell source largely because of the convenience of peripheral collection techniques. PBSC harvesting by single or multiple leukapheresis procurements avoids the risks associated with multiple marrow aspirations and general anesthesia and shortens donor recovery time. PBSCs are mobilized in the donor using G-CSF for several days before peripheral blood collection. This increases circulating CD34 + stem cell concentrations approximately 16-fold from baseline and easily compensates for the low baseline counts. The total numbers of T cells, monocytes, and NK cells contained in a PBSC allograft are more than 10 times higher than those in a blood marrow allograft. Despite this, there is no difference in the incidence of acute GVHD in patients who receive PBSC compared with blood marrow allografts. However, it is thought that the increase in T cells in PBSCs compared with blood marrow allografts results in an increased incidence of chronic GVHD in recipients of PBSC allografts. Migration of blood marrow–derived stem cells to the marrow niches is slightly better than PBSCs. However, neutrophil and platelet engraftment is significantly faster using PBSCs. Additionally, recipients of PBSC transplants have higher naïve and memory T cells as well as T-cell proliferative responses to mitogens at 1 and 11 months after transplant compared with blood marrow transplant recipients. Although there is no difference in outcomes in adult HSCT recipients, some studies suggest pediatric patients who receive PBSC, compared with blood marrow grafts, have higher rates of treatment-related mortality, treatment failure, and mortality related to increased incidence of GVHD. ,
Umbilical cord blood.
UCB has also been used as a stem cell source for more than 30 years. Umbilical cord stem cells allow for an increased level of HLA disparity; therefore cord blood transplantation (CBT) is an option for alternative hematopoietic stem cells when there are no available related or unrelated donors. This can be particularly important for racial or ethnic minorities who have limited URDs available in the National Marrow Donor registry. Furthermore, registry searches and screening can take several weeks. UCB has the additional advantage of immediate availability of cells and thus can be useful for urgent transplantation of certain malignant diseases. CBT has the advantage of lower rates of chronic GVHD compared with recipients of PBSC products. Although UCB contains significantly higher absolute numbers of T, NK, and B lymphocytes, most of the UCB T-cells are naïve T cells, resulting in prolonged time to engraftment, lack of transferred T-cell memory, and a high incidence of opportunistic infections, particularly in the first 3 to 4 months after transplant. Early transplant-related nonrelapse mortality in CBT is primarily due to infectious complications, presumably related to the relative delay in neutrophil engraftment and CD8 + immune reconstitution. Mortality from opportunistic infections in CBT can be predicted by age, CMV serostatus, HLA mismatch, and lower graft cell dose. In addition to the high mortality associated with opportunistic infections, CBT is also complicated by an increased incidence of graft failure and relapse. T-cell reconstitution is dependent on thymopoiesis to produce long-term memory cells. Markers of thymopoiesis (recent thymic immigrants, TRECs, CD4 + CD454RA+ T cells, and TCR repertoire diversity) are associated with CBT outcomes such as infections, disease, relapse, and overall survival. Although UCB is enriched for hematopoietic stem cells, it is limited by the absolute quantity of stem cells that can be collected from a single donor. Given the association between stem cell dose and time to neutrophil engraftment, the use of double cord blood transplantation (dCBT), from two unrelated, partially matched grafts, has been used as a means to increase stem cell dose. After dCBT one of the grafts dominates long-term reconstitution while the other mediates short-term engraftment. Perhaps because of the increase in stem cell dose, there may be an increased risk of GVHD, and therefore, a benefit of graft-versus-leukemia (GVL) effect from dCBT. However, single-unit CBT was associated with better platelet recovery, a lower risk of GVHD, and a significant improvement in survival after dCBT compared with single-unit CBT has not been confirmed. Despite the advances achieved with increasing cell dose in dCBT, there still remains a gap in the time to engraftment compared with blood marrow and PBSC grafts and this delay in engraftment is associated with an increased early transplant-related mortality, likely related to infectious complications. ,
Stem cell dose
After stem cell collection, via either bone marrow harvest or peripheral blood apheresis, grafts are evaluated to determine the estimated stem cell dose. The total nucleated cell dose and CD34 + cell dose are important factors contributing to the rate of engraftment. However, the dose required for rapid and stable long-term engraftment varies depending on the method of measurement and the source of stem cells. The dose of hematopoietic stem cells infused affects the rates of hematopoietic recovery after HSCT. Specifically, increased stem cell dose decreases the time to neutrophil engraftment. Despite improving the time to engraftment, it is unclear if increased stem cell doses improve overall immune reconstitution; however, the receipt of higher total nucleated cell doses has been associated with increased survival and decreased relapse. However, the benefits of increasing stem cell dose need to be balanced against the associated increased risk of acute GVHD. Stem cell doses are also limited with certain types of stem cell sources, such as UCB.
Pretransplant conditioning
The conditioning (or preparative) regimen is designed to provide myeloablation of the recipient marrow to allow for donor engraftment and immunosuppression to prevent rejection. Conditioning regimens may use chemotherapeutic drugs, serotherapy (antithymocyte globulin or alemtuzumab), and/or total body irradiation. The ideal conditioning regimen is based on clinical judgment that accounts for underlying disease, comorbidities, disease status, and donor and graft source. Conditioning regimens can be categorized as myeloablative, reduced intensity, or nonmyeloablative. Although the definitions are somewhat debated, myeloablative regimens consist of a single agent or combination of agents expected to destroy the hematopoietic cells in the bone marrow and produce profound pancytopenia. Reduced intensity or nonmyeloablative conditioning regimens consists of agents that do not fully eliminate the possibility of host hematopoietic recovery.
The use of myeloablative conditioning regimen results in long-lasting, likely irreversible, destruction of hematopoietic cells in the bone marrow and potential delays in immune reconstitution through thymic damage. The thymus is responsible for the generation of a diverse naïve T-cell receptor repertoire. After HSCT, the thymus is occupied by hematopoietic progenitors, which will later diversify and acquire an adaptive cellular immune response. Although myeloablative, reduced intensity, or nonmyeloablative regimens can damage the thymus, it is not known if the degree of damage varies by conditioning intensity.
Reduced intensity conditioning regimens have been developed as a means to achieve engraftment, allow for graft-versus-tumor effect, and limit chemotherapy-related toxicities. These regimens contain a varying degree of myelosuppression and immunosuppression and may include both chemotherapy, serotherapy, and radiation. One of the hypothesized advantages of reduced intensity conditioning is that it might lend to better immune reconstitution after transplantation owing to less damage of the thymus, allowing regeneration of naïve T cells derived from donor HSCs and perhaps also owing to proliferation of immunologically competent host T cells that survive the conditioning regimen. However, studies have shown conflicting findings and are difficult to interpret given the variability in protocols. Furthermore, donor lymphocyte infusions (DLIs) are frequently used after reduced intensity stem cell transplantation and may contribute to earlier recovery of some immune function via the transfer of memory T cells. Conversely, DLIs may induce GVHD, leading to further immune suppression.
Recipient- and donor-specific factors
Donor age.
As the immune system ages, there is increased susceptibility to infections and cancer, decreased responsiveness to vaccines, and increased incidence of autoimmune disorders. The mechanisms underlying immunosenescence (the changes seen with an aging immune system) are complex and still being explored. Hematopoietic stem cells from aged donors have reduced engraftment capacity and potential for reconstitution. Consequently, increased donor age, even as young as 36 years, can affect HSCT outcomes. Additionally, increasing donor age seems to be associated with a defect in hematopoietic stem cell function that skews that lineage potential away from lymphoid and toward myeloid precursors.
The effect of donor age was best demonstrated in a retrospective analysis from the Center for International Blood and Marrow Transplant Research of more than 11,000 unrelated transplants performed from 1988 to 2011 that evaluated the effects of various donor characteristics (e.g., age, sex, CMV serologic status, ABO compatibility, race, and parity) on recipient outcome. After adjustment for patient disease and transplant characteristics, age and donor-recipient HLA match were the only donor traits significantly associated with overall survival. For every 10-year increment in donor age, there was a 5.5% increase in the hazard ratio for mortality. Older donor age was also associated with an increase in acute, but not chronic, GVHD. Other studies have found that younger donors are also associated with lower incidences of serious complications, including secondary graft failure, posttransplant lymphoproliferative disease, obstructive lung disease, and relapse after allogeneic transplantation.
Recipient age.
Age-related decline of the immune system’s ability to regenerate a lymphocyte pool is an obstacle in stem cell transplantation, leading to increased susceptibility to infections and decreased efficacy of vaccines. Thymic involution and subsequently reduced exportation of naïve T cells is the most well described age-related change. The thymic microenvironment is in slow, but constant, change and eventually involutes with age. After total body irradiation and chemotherapy for conditioning there is significant damage to the thymic epithelial microenvironment, which results in reduced T-cell development. Recovery of the thymic function after HSCT is largely dependent on the age of the recipient. In young patients, the long-term recovery of thymic function is unaffected and the epithelial compartment eventually recovers from chemotherapy. In comparison, thymic damage caused by cytoreductive conditioning can be particularly detrimental in older individuals whose thymus has already undergone significant involution. Although thymic aging can be observed as early as 1 year of age, significant impacts of aging on immune reconstitution are not apparent until after puberty. Notably, the adult thymus still appears capable of regeneration at least up to middle age.
Sex and parity.
Sex and parity are the most controversial of factors that can potentially affect stem cell transplant outcomes, especially when female donors are used for male recipients. This risk is thought to be due to the various Y chromosome–encoded T-cell epitopes, the HY minor histocompatibility antigens, the presence of which on male host tissues can be recognized by female donor T cells. This effect may be magnified in parous female donors to male recipients, who have developed memory lymphocytes against HY histocompatibility alloantigens. However, some nulliparous women also have allosensitization to HY antigens (via unclear mechanisms). A large Center for International Blood and Marrow Transplant Research database multivariate analysis evaluating the effects of donor or recipient sex and parity showed no effect on the risk of acute GVHD; however, sex and parity were significantly associated with chronic GVHD. For male recipients, nulliparous and parous female donors conferred an increased risk of chronic GVHD. For nulliparous and parous female recipients, parous female donors also significantly increased the risk of chronic GVHD. , There is no association between sex or parity and survival, relapse risk, or transplant-related mortality.
Cytomegalovirus status.
CMV, a virus that establishes lifelong persistence, can reactivate during and after HSCT in the period after receiving conditioning while awaiting immune reconstitution. CMV has a bidirectional relationship with immune reconstitution. Delays in immune reconstitution lead to increased risk of CMV infection; however, CMV itself may have immune suppressive effects that can further delay immune reconstitution.
During the early posttransplant period when patients are most immunocompromised, seropositive recipients are at risk of CMV reactivation and increased transplant-related mortality. The risk is highest with CMV-seropositive recipients who receive grafts from CMV-seronegative donors; therefore CMV-seropositive donors are always preferred. Additionally, both UCB transplantation and haploidentical HSCT result in delayed immune reconstitution, and therefore pose additional risks of CMV reactivation and infection. The use of newer TCD methods (such as α/β TCD) and reduced intensity conditioning can somewhat mitigate the increased risk of CMV infection after haploidentical stem cell transplantation. CMV reactivation most often occurs in the first 100 days after transplant, but with concomitant chronic GVHD or in the setting of haploidentical transplantation, reactivation can occur much later. Studies suggest that recovery of both CMV-specific CD4 + and CD8+ T cells is essential for controlling CMV after HSCT. Control of CMV is dependent on expansion of CMV-specific CD8 + cytotoxic T lymphocytes (CTLs). Evaluation of CTL function after allogeneic HSCT revealed that 50% of patients exhibited a detectable CMV-specific CTL response by 3 months after allogeneic transplantation. Restoration of CTL response appeared to be dependent on CD4 + recovery. The factors influencing the recovery of CMV-specific CD4 + and CD8 + function after HSCT are poorly understood, but the use of high-dose steroids and type of stem cell source (bone marrow) have been associated with impaired CD4 + and CD8 + function 3 months after transplantation.
Once CMV reactivates, the infection itself can further delay immune reconstitution and repopulation of a more diverse T-cell repertoire. One hypothesis for CMV infection to actively interfere with immune reconstitution is via infection of bone marrow stromal cells and reducing the homing of transplanted hematopoietic stem cells to bone marrow stroma, leading to graft failure. Conversely, a unique association exists between CMV infection in transplant recipients with acute myelogenous leukemia (AML), wherein CMV reactivation is associated with protection from leukemic relapse, likely caused by the CMV-driven expansion of donor-derived memory-like NK cells and γ/δδ T cells.
Graft-versus-host disease.
In allogeneic transplant recipients, the presence of clinically significant GVHD is the most influential factor affecting the timing of immune reconstitution. Acute GVHD is an immunologic response against the host immune system, tissues, and organs and is primarily mediated by alloreactive donor T cells. Acute GVHD occurs in 20% to 60% of patients who receive allogeneic HSCTs and substantially contributes to transplant-related nonrelapse mortality. Development of acute GVHD is primarily influenced by HLA or less likely gender mismatches, the intensity of the conditioning regimen, CMV reactivation, and the stem cell source. The risk of GVHD can be partially mitigated by good donor selection, choice of conditioning regimen, TCD methods, and pharmacologic prophylaxis. Donor selection should always aim to minimize HLA disparity, with the possible exception of malignant diseases in which GVL responses (discussed later) may play a role, with preference for matched sibling donors, and perhaps male donors and nulliparous donors. With regard to conditioning regimens, myeloablative regimens are associated with a higher risk of GVHD than reduced intensity conditioning regimens, likely owing to increased tissue injury, particularly in the gastrointestinal tract. A certain graft source may also be preferred depending on these factors. For example, given the decreased risk of GVHD with bone marrow or UCB, bone marrow or CBT may be preferred in the setting of myeloablative conditioning, whereas peripheral blood stem cells are preferred in the setting of reduced intensity conditioning.
Impact of graft-versus-host disease prophylaxis on immune reconstitution.
Prophylaxis of acute GVHD is centered around immunosuppression of the donor T cells, either pharmacologically or via ex vivo TCD. Pharmacologic prophylaxis does not have as profound an impact on immune reconstitution compared with ex vivo TCD methods; therefore pharmacologic prophylaxis may be preferred to TCD in the appropriate setting. GVHD pharmacologic prophylaxis regimens varies significantly among institutions but include agents such as antithymocyte globulin, alemtuzumab, cyclophosphamide, methotrexate, cyclosporine, tacrolimus, mycophenolate, and corticosteroids. Combination therapy, using more than one agent, is typically used as it is associated with a reduction in risk for acute GVHD compared with the use of single agents. Generally, the goal of GVHD prophylaxis is to maintain immunosuppression for the first 3 months after transplantation, but this duration may range between 4 weeks to 6 months after transplantation depending on the regimen used. In patients without GVHD, withdrawal of immunosuppression is associated with a reduced risk of relapse during the first 18 months; therefore shorter durations of GVHD prophylaxis are preferred for malignant disease.
When antithymocyte globulin (ATG) or alemtuzumab (anti-CD52) is administered to the recipient in the pretransplant period, this is referred to as serotherapy or in vivo TCD. Serotherapy is considered instrumental in the prevention of rejection of the graft, especially from mismatched donors; however, owing to their long half-lives, ATG and alemtuzumab continue to be present at the time of donor product infusion and eliminate donor T cells, thereby offering prevention of acute GVHD. Despite the beneficial effects on prevention of graft rejection and GVHD, serotherapy adversely causes immunosuppression, delay in immune reconstitution, and increased risk of viral infections and reactivations, particularly with adenovirus. Alemtuzumab has a longer half-life (15 to 21 days) than ATG (4 to 14 days) and therefore is associated with more prolonged T- and NK-cell recovery compared with ATG.
Ex vivo TCD is an alternative approach to pharmacologic agents for GVHD prevention that requires manipulation of the donor product prior to administration to the recipient. Although the use of TCD methods may reduce the risk of GVHD in haploidentical stem cell transplant recipients, depending on the technique, it may be associated with significant risks, including delayed immune reconstitution, infectious complications and increased risk of graft failure and relapse. Ex vivo TCD includes methods such as CD8 + cell depletion, CD3 + /CD19 + cell depletion, TCR α/β T cell with CD19 + cell depletion, or naïve TCD with or without T-cell add-back. Initial trials of ex vivo TCD using monoclonal antibodies admixed with the cells was associated with high risk of GVHD, leading to the additional treatment of T cells with complement or immunotoxins to eliminate T cells from the graft, which resulted in a 10% to 20% reduction of GVHD risk without pharmacologic prophylaxis. However, stringent TCD has been associated with increased risk of graft failure because donor lymphocytes, including both T cells and NK cells, are important mediators in engraftment. Therefore TCR α/β TCD methods that allow for repletion of donor γ/δ T cells and NK cells may help facilitate engraftment and prevent primary rejection. Finally, the use of CD34 + positive selection is an approach that can exclude lymphocytes or immunologic components from the donor product that might be implicated in the pathogenesis of GVHD. This method has demonstrated substantial reductions in both acute and chronic GVHD while maintaining good disease control in patients with acute leukemia and myelodysplastic syndrome. Despite concomitant use of myeloablative conditioning regimens, CD34 + selection shows good short-term (1 year) and longer-term (>1 year) toxicity outcomes, nonrelapse mortality, and overall survival.
The mechanisms that predispose allogeneic stem cell transplant recipients to chronic GVHD are poorly defined; however, the most consistently documented risk factor for chronic GVHD is a history of acute GVHD. At this time, there is no specific immunoprophylaxis used to prevent chronic GVHD.
Impact of graft versus host disease on immune reconstitution.
Although the pharmacologic agents administered to prevent or treat GVHD are a significant source of immune suppression, clinicians need to also recognize that GVHD in and of itself can result in delayed immune recovery. This is because acute GVHD represents an immunologic response against the host tissues, organs, and immune system, primarily mediated by alloreactive donor T cells.
Both the bone marrow, important for the development of hematopoietic progenitors, and the thymus, important for the maturation of hematopoietic precursors and T-cell development, are sites of alloreactivity during GVHD. Massive apoptosis and release of cytokines results in decrease in thymic output, delayed recovery of CD4+ T cells, and restricted T-cell receptor repertoires. Thymic epithelial cells are a direct target of alloreactive interferon γ–secreting donor T cells, which can lead to failure of donor-derived progenitors to differentiate via the classical central pathway. The severity of acute GVHD-associated T-cell hypoplasia implies that GVHD abrogates not only thymic but also extrathymic T-cell reconstitution. After initial brisk proliferation of alloreactive T cells, antihost T cells undergo massive Fas-mediated apoptosis and lysis of grafted mature T cells. Despite this, in younger patients (<25 years of age) with acute GVHD, thymic function generally recovers almost completely at 1 year and post-thymic T-cell lymphopoiesis typically resumes with resolution of GVHD and withdrawal of immunosuppression. Owing to the severe and brisk destruction of lymphocytes and lymphocyte progenitors, advanced GVHD is the most significant independent predictor of subsequent (second, third, or fourth) infections. In addition to the impact on T-cell recovery, acute GVHD can have significant compromise of the skin and mucosal barriers of the alimentary tract that predispose to infection. The treatment of patients with acute GVHD with corticosteroids or other immunosuppressive drugs further increases the risk of infection.
By definition, chronic GVHD (cGVHD) presents after 100 days posttransplantation as a chronic inflammatory and sclerotic autoimmune-like condition that most frequently affects the skin, oral mucosa, liver, eyes, and gastrointestinal tract. The immunologic mechanisms underlying cGVHD are complex and differ from mechanisms underlying acute GVHD. Although donor-derived T cells are still considered to be the preeminent mediators of cGVHD alloreactivity, aberrant B cells clearly play a significant role in promoting autoimmunity and inflammation. The enhanced activity of T follicular helper cells in cGVHD appears to play a key role in the aberrant B-cell activity and the resulting autoimmune-like features of cGVHD. It is thought that the alloreactivity and massive lymphoid apoptosis observed in acute GVHD might be responsible for the occurrence of autoimmunity in cGVHD. This delay in immune reconstitution is primarily through thymic destruction and, in contrast to the damage seen in acute GVHD, is likely irreversible. Patients with cGVHD are found to have low TRECs and shortening of telomere length, a measure of T-cell replicative capacity. Furthermore, HSCT recipients in whom cGVHD develops are at increased risk of infections related to encapsulated organisms (particularly Pneumococcus), likely as the result of splenic dysfunction. This broad and profound immunologic dysfunction confers susceptibility to serious, often life-threatening, infections. In fact, chronic GVHD is the leading cause of late treatment-related deaths among HSCT recipients, and greater severity of cGVHD correlates with worse outcomes.
Graft-versus-leukemia effect.
With HLA mismatched transplantation, donor T lymphocytes and NK cells may recognize neoplastic cells as foreign due to the expression of epitopes unique to the host. Cytotoxic T lymphocytes and NK cells subsequently become activated, lysing such cells. The T lymphocyte GVL or graft-versus-tumor effect is similar to that underlying GVHD. The first direct evidence of a pronounced antileukemic effect of GVHD was found in patients with AML with acute and/or chronic GVHD who had decreased relapse compared with patients with AML without GVHD. Patients with AML who received syngeneic (twin sibling) grafts have an increased risk of relapse compared with allograft recipients without GVHD. Additionally, recipients of T-cell replete allografts have lower rates of relapse in the absence of GVHD compared with recipients of T-cell–depleted grafts. However, this difference may not necessarily translate into prolonged disease-free survival in view of the morbidity and mortality associated with chronic GVHD. Although cGVHD is associated with fewer relapses of leukemia, the severity of cGVHD does not further decrease the incidence of relapse. So, there may be a benefit to a mild degree of GVHD in patients with leukemia; however, avoidance of severe GVHD is still preferable. GVHD prophylaxis may be effective; however, an important concern is that such therapy would diminish the GVL effect. Furthermore, the association of GVHD with risk of relapse changes over time and HSCT recipients with GVHD have decreased risk of relapse after, but not before, 18 months after transplant. The GVL effect could potentially be enhanced to prevent relapse. In patients without GVHD, withdrawal of immunosuppression might help to prevent relapse during the first 18 months after transplant but is likely not effective after 18 months.
Despite this, GVL effects can be found independent of clinically significant GVHD and the immunologic mechanisms likely differ from those that underlie GVHD. It is unclear whether GVL in the setting of absent or mild GVHD has an impact on the immune system. However, any impact appears to be relatively mild, given the lack of increased transplant-related mortality in patients with only mild GVHD. These findings have led to the investigation into other immunologic mechanisms involved in GVL effects, such as NK cells, killer cell immunoglobulin-like receptors (KIRs), and γ/δ T cells. KIRs are a particularly important moderator in the GVL effect. KIRs are surface receptors present on NK cells and a subset of T lymphocytes. Mismatching of the KIR ligand in the GVHD direction appears through NK-cell and cytotoxic T-cell activation, which result in a lower risk of relapse after allogeneic HSCT. This effect appears to be more evident in haploidentical transplantation using grafts depleted of T cells and in UCB transplantation. Other mechanisms to augment the GVL effect include the use of donor lymphocyte infusions to induce sustained remissions. In the future, GVL effects may be enhanced through vaccine therapy or adoptive transfer of selective T cells to improve a portion of the immune response involved in GvL.
Assessment of immune reconstitution
There is no single marker for assessment of immune reconstitution after HSCT. This is primarily because recovery of a specific immune component of interest may require assessment of multiple laboratory values and will depend on the time period after HSCT under consideration. For example, neutrophil engraftment, assessed by frequent measurement of neutrophil counts, is classically used as a measure of innate immune system recovery. This is a common focus during the immediate early post-HSCT period. However, even when a patient is said to have achieved neutrophil engraftment (e.g., >500 neutrophils/μL), this result does not actually measure donor cell engraftment, as autologous reconstitution of neutrophils is possible after most conditioning regimens, nor does it inform on other recovery of other components of the innate immune system. Innate immune reconstitution can be further evaluated by measurement of NK cells. Recovery of the adaptive immunity is often of interest later after transplant and can include assessment of T-cell immunity via enumeration of T-cell subsets (CD3 + , CD4 + , CD8 + , and CD4 + CD45 + RA) and lymphocyte proliferative response to phytohemagglutinin. Although neutrophils are often measured frequently in the immediate post-HSCT period, the timing and form of measurement to assess recovery of other innate and adaptive function is variable by institution. Furthermore, it is not clear which level of a given test constitutes evidence of true reconstitution for a specific immune component.
Clinical research studies have used several markers at different time points of immune reconstitution, including absolute lymphocyte count, absolute CD4 + count, CD4/CD8 ratio, T-cell subset testing by flow cytometry, Ig levels, rise in antigen-specific antibody titer after vaccination, lymphocyte mitogenic responses, quantification of TRECs, and T-cell receptor spectratyping or deep sequencing to assess the T-cell repertoire. Results from these studies have informed some general conclusions about laboratory values and general correlates of protection. For example, lymphocyte count 1 month after allogeneic HSCT is associated with better outcomes, including lower transplant-related mortality, higher relapse-free survival, and improved overall survival. Additionally, a CD4 + T-cell count below 200/μL at 3 months has been shown to be associated with increased infections, increased nonrelapse mortality, and decreased overall survival. However, there remains an absence of clear guidance as to which immune function studies are clinically informative—and even if they are informative—and exactly when should they be performed. Because of the paucity of such data, institutions often develop their own approach for what laboratory tests to order, when to order them, and how to use the results for clinical decision making. This ultimately results in significant interinstitution variability. More data are needed to define the impact of different testing approaches on clinical outcomes so that centers can order these studies judiciously and harmonize their testing approaches.
Improving immune reconstitution after HSCT
Hematolymphopoiesis occurs in association with a complex network of cell types found in the bone marrow stroma, including nonhematopoietic (fibroblasts, adipocytes, and endothelial cells) and hematopoietic cells (macrophages and T-cells). Progenitor cell growth and differentiation depend on their interaction with stromal cells. Outside the bone marrow, lymphoid progenitors emigrate to the thymus to proliferate, mature, and differentiate. Several strategies have been proposed to enhance immune reconstitution after HSCT ( Table 2.2 ), including cellular therapies, cytokines and hormones, sex steroid ablation, or enhancement of thymic tissue including the use of IL-7, thymosin α 1 , growth hormone, keratinocyte growth factor, and sex steroid ablation. Of these, IL-7 has been most extensively studied in experimental and clinical trials. IL-7 acts directly on T-lymphoid precursors as a pro-lymphopoietic cytokine and has been shown to enhance thymopoiesis. A phase I trial of recombinant IL-7 in 12 adults undergoing T-cell–depleted allogeneic HSCT suggested that this approach improved T-cell recovery and T-cell repertoire diversity without increased GVHD or other toxicities. Keratinocyte growth factor is a thymic epithelial cell (TEC) mitogen that stimulates proliferation and, when given before pretransplant conditioning, reduces TEC injury. Although keratinocyte growth factor has differential responses in various subsets of TECs, its efficacy can be enhanced in murine models by using p53 inhibition to restore cortical and medullary TECs and improve thymic function after HSCT. Estrogen and testosterone have been implicated in the regulation of thymopoiesis, B-cell lymphopoiesis, and early lymphoid precursors. Androgen inhibition with leuprolide has been shown to reverse age-related thymic involution in animal models. Keratinocyte growth factor and androgen blockade may work in combination to protect TECs from conditioning-induced damage. These mechanisms have been studied in murine models; however, the effects of keratinocyte growth factor and androgen blockage on immune reconstitution in human stem cell recipients is not yet elucidated.
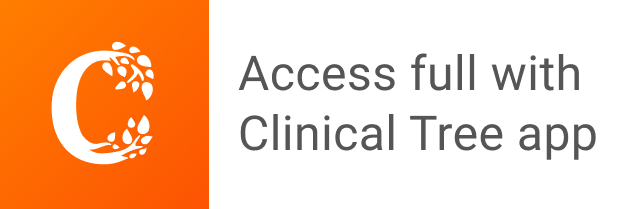