Fluorescent In Situ Hybridization and Other Molecular Techniques in the Diagnosis of Cutaneous Lymphomas
Andrea L. Salavaggione
Alejandro A. Gru
FLUORESCENT IN SITU HYBRIDIZATION
In situ hybridization (ISH) allows for detection and localization of a specific DNA or RNA sequence directly in tissue sections by use of labeled complementary DNA or RNA probes. In contrast to immunohistochemistry (IHC), which analyzes surface, cytoplasmic, or nuclear expression of specific proteins, ISH is able to identify specific RNA or DNA sequences.1,2 At the early application of ISH in the 1960s, the probes were labeled with radioactive nucleotide analogs.3 The drawback of radio-labeling included instability of the probes, limited resolution, long exposure times, and risks associated with the handling of radioactive agents. This problem was overcome in the 1980s with the introduction of fluorochromes to make fluorescent, rather than radioactive, ISH (fluorescent in situ hybridization [FISH]).4
The advantages that FISH has over conventional cytogenetic methods (conventional karyotype, G-banding) are the lack of dependence on live cells and need of metaphases. FISH can be performed on interphase chromosomes of tissue samples in formalin-fixed paraffin-embedded (FFPE) tissue. Moreover, it has a much higher resolution allowing the identification and localization of short DNA or RNA fragments and the detection of numerical aberrations within single cells. One of the disadvantages is the need for probes that are at least 40 to 50 kilobase (kb) in length, as smaller probes are too difficult to identify in tissue sections. FISH has an extraordinarily high specificity. False-positive rates approximate 1% to 5% because of special co-localization in FFPE tissue.1 One of the limiting factors in the utility of FISH is the restricted number of probes available for specific molecular diagnostic detection. One of the newer inventions in ISH is the use of chromogen-labeled probes (chromogen in situ hybridization [CISH]). CISH can be applied to FFPE allowing an easier approach with good morphologic correlation and cytogenetic findings.5 Perhaps, the most common use of CISH techniques includes analysis of κ and λ cytoplasmic light chain restriction, mRNA detection for EBV-specific sequences (Epstein–Barr encoded RNA [EBER]), HPV subtyping, and detection of HER2 amplification in biopsies of patients with breast cancer. In addition to the maturation of the technique, its applicability broadened from the detection of single nucleic acid targets to multiple targets in a single assay by the simultaneous use of multiple probes, each labeled with a spectrally distinct fluorochrome evaluated by a computer-based ratio analyses of the resulting color combinations and intensities. The discrimination of many more targets than the number of available, spectrally resolvable fluorochromes can be achieved using combinatorial labeling or ratio labeling. These different labeling strategies allow the simultaneous visualization of 24 human chromosomes in a single hybridization. The technologies using these approaches include M-FISH, SKY, and COBRA.6,7
Two principal types of probes are used to detect chromosomal rearrangements: (1) “split-apart” or “break-apart” probes labeled in different colors that bind to regions on either side of a gene breakpoint; and (2) “co-localizing” or “dual-fusion” probes that label each gene in a single color giving fused signals in a cell with a specific translocation.2 Both types of probes may also reveal gene amplification, and centromeric FISH probes to allow the detection of aneuploidy are also available (Fig. 9-1).
COMPARATIVE GENOMIC HYBRIDIZATION
Comparative genomic hybridization (CGH) is a FISH-based technology that started in the early 1990s.8 Array CGH (aCGH) allows for the assessment of genetic gains and losses over the whole genome. The total genomic DNA is marked with a particular fluorochrome (e.g., green), and the DNA from a normal reference is labeled with a different dye (e.g., red). Red and green probes are then hybridized competitively to unrelated metaphase chromosomes, which function as the reading device. The fluorescence balance between the sample and reference dyes is evaluated quantitatively. In the case of a normal genetic allocation between test and reference sample, the resulting fluorescence will be a 50/50% balance between green and red (yellow); gains (gene amplification) and losses (deletion) will be more intensive for green and red, respectively. With CGH performed on metaphase chromosomes, copy number changes at the size of several megabases can be detected. The precision to detect smaller genomic changes down to the length of kilobases (usually approximately 80 to 120 kb) has been achieved by exchanging the metaphase chromosomal-based reading device to a microarray platform (aCGH). With a microarray, a high number of specific and defined DNA sequences can be screened for genetic aberrations (Fig. 9-2). As CGH measurements always represent an average value over the entire cell population analyzed, copy number changes must be present in a substantial proportion of cells (approximately 30% to 50%) in order to be identifiable.9 Therefore, specific aberrations seen in a small proportion of cells will be missed by aCGH. In addition, CGH does not allow the detection of balanced chromosomal translocations, inversions, and point mutations. CGH is also cost intensive, expensive depending on the skills of a laboratory personnel and it is hard to establish in a new laboratory. aCGH has been clinically used in the diagnosis of melanocytic lesions more extensively.10,11,12
G-BANDING—CONVENTIONAL KARYOTYPE
G-banding is a relatively straightforward and inexpensive method to screen the entire genome for numerical or structural chromosomal aberrations in viable tissues.13 Its major restriction represents the need for viable metaphases in live cell suspensions. This requires viable tumor with a significant proliferative rate. The metaphase spreads are stained with Giemsa dye revealing a distinctive banding pattern along each chromosomal arm. As each band spans a huge part of a chromosome, subtle translocations, deletions, or insertions will produce a normal banding pattern and can therefore not be detected by this method. The addition of FISH to the conventional metaphases is routinely used in the diagnosis of specific hematologic malignancies (Fig. 9-3).
A comparison of FISH, aCGH, and conventional karyotyping is summarized in Table 9-1. We will discuss the use of these techniques in the diagnosis of cutaneous lymphoproliferative disorders.
Table 9-1 Comparison of Common Cytogenetic Techniques Available in the Diagnosis of Lymphomas | ||||||||||||||||||||
---|---|---|---|---|---|---|---|---|---|---|---|---|---|---|---|---|---|---|---|---|
|
MYCOSIS FUNGOIDES AND SÉZARY SYNDROME
Chromosome abnormalities, mostly complex karyotypes, are seen in about 50% of patients with mycosis fungoides/Sézary syndrome (MF/SS), and there have only been a few instances of recurrent rearrangements.14 Approximately 47% of cases have an abnormal karyotype. The most frequent abnormalities involve chromosome 10; followed by chromosome 6; chromosomes 3, 7, 9, 17, and 19; chromosomes 1 and 12; and chromosomes 8, 11, and 13. Most abnormalities were structural. Recurrent rearrangements included deleted chromosomes 6 and 13, and recurrent breakpoints at 1p32-36, 6q22-25, 17p11.2-13, 10q23-26, and 19p13.3. A pseudodicentric translocation between the short arms of chromosomes 8 and 17, confirmed by dual-color FISH and interpreted as psu dic (17;8)(p11.2;p11.2), has been noted in a total of three cases. Unbalanced translocations involving chromosomes 4 and 14 have also been noted in SS.15 Karenko et al.16 showed clonal deletions or translocations with a break point in 12q21 or 12q22 in five of seven consecutive SS patients and a clonal monosomy in the sixth patient. The translocation involved the NAV3 gene. With locus-specific FISH, NAV3 deletions were found in the skin lesions of 4 of 8 (50%) patients with early MF (stages IA–IIA) and in the skin or lymph node of 11 of 13 (85%) patients with advanced MF or SS. NAV3 may contribute to the growth, differentiation, and apoptosis of cutaneous T-cell lymphoma (CTCL) cells as well as to the skewing from TH1-type to TH2-type phenotype during disease progression. A finish study showed that the presence of NAV3 deletion correlated with poor response to retinoid therapy.17 Others have shown recurrent deletions of 10q and 17p and amplifications of 8q and 17q, with robust evidence implicating deletions of TP53 and CDKN2A and amplification of 8q containing MYC.18,19,20,21
CGH analysis showed chromosome imbalances (CIs) in 19 of 34 CTCL cases (56%). The most frequent losses involved chromosomes 1p (38%), 17p (21%), 10q/10 (15%), and 19 (15%), with minimal regions of deletion at 1p31p36 and 10q26. The commonly detected chromosomal gains involved 4/4q (18%), 18 (15%), and 17q/17 (12%).22 A subsequent study in patients with SS showed 12 of 28 cases with clonal chromosome abnormalities (43%) by conventional cytogenetics.23 Seven cases had aberrations affecting chromosomes 1 and 17; five demonstrated rearrangement of chromosomes 10 and 14; and four presented with an abnormality of 6q. Multiplex-fluorescence in situ hybridization (M-FISH) revealed complex karyotypes in 6 of 17 cases (35%). BCL2 deletion and amplification of JUNB have also been noted in cases of MF and SS.24,25 Dysregulations of the CCND1 and RB1 genes have also been noted in CTCL.26 The presence of translocations involving the T-cell receptor (TCR) loci (a frequent phenomenon in many systemic T-cell lymphomas and mature leukemias) is rare in CTCL.27 Utikal et al.28 also revealed numerical gains of the HER2 gene in SS, comparing to the number of chromosome 17 centromeres. They also showed increased expression of the protein by IHC, suggesting a possible role for Herceptin in the treatment of a subset of such patients. The IRF4 gene rearrangement, typical of cutaneous anaplastic large cell lymphoma (C-ALCL), is not seen in transformed MF.29
Major advances have been published recently in relation to the mutational landscape of MF and SS, in addition to the discovery of particular molecular rearrangements.30,31,32,33,34 Choi et al.30 found frequent deletions in chromatin-modifying genes (ARID1A [62.5%], CTCF [12.5%], and DNMT3A [42.5%]). RB1 was deleted in 25% of samples. CARD11 and JAK2 amplification were seen in 22.5% and 12.5% of cases, respectively. MYC amplification was noted in 42.5% of samples. Da Silva Almeida et al.31 identified a median of 21 copy number alterations per sample (range, 0 to 56) in SS, with characteristic recurrent gains in chromosome 7 (5/25, 20%), 8q (13/25, 52%), and 17q (2/25, 8%), as well as recurrent deletions involving tumor-suppressor genes in 17p13.1 (TP53; 13/25, 52%), 13q14.2 (RB1; 4/25, 16%), 10q23.3 (PTEN, 5/25; 20%), and 12p13.1 (CDKN1B; 5/25, 20%). Kiel et al.32 showed numerous (n = 42) fusion genes including TPR-MET, MYBL1-TOX, DNAJC15-ZMYM2, and EZH2-FOXP1, which, albeit not recurrent, could contribute to the pathogenesis of SS. Ungewickell et al.33 found structural variation events (excluding copy number gains) in pathways related to T-cell survival and proliferation in 11% of patients with MF or SS. Interestingly, the structural variants were largely mutually exclusive with the TNFR2 alterations. The structural variants included NFKB2 gene truncations in 5% (4/73) of cases with the deletion of a region whose loss is known to generate a truncated p100 protein with predicted proteasome-independent NF-κB2 nuclear localization, as well as a deletion involving TRAF3 that would also be expected to increase noncanonical NF-κB signaling. A recurrent CTLA4–CD28 fusion was also discovered.
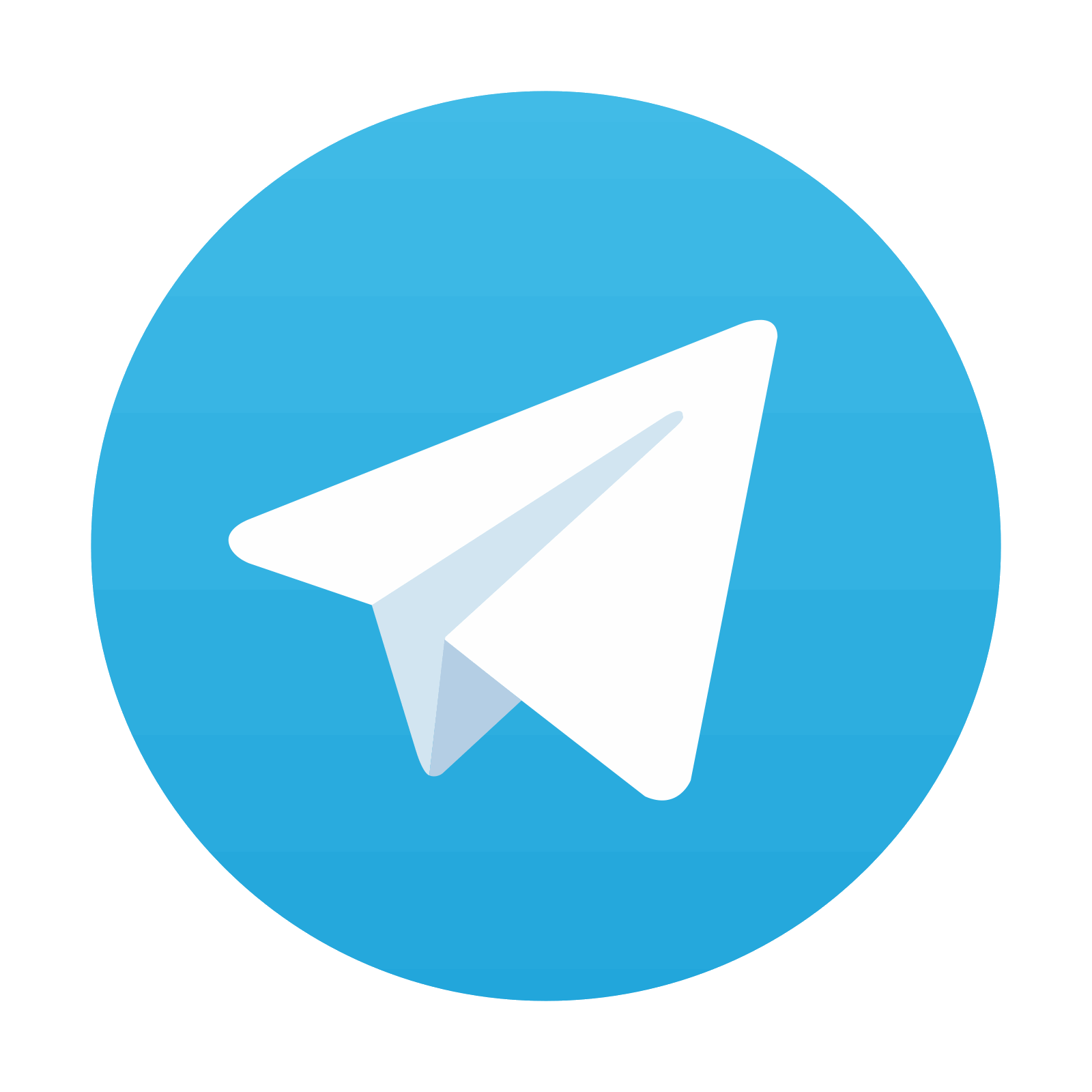
Stay updated, free articles. Join our Telegram channel
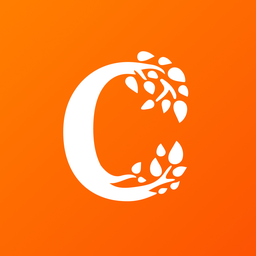
Full access? Get Clinical Tree
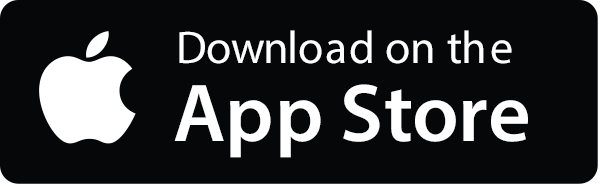
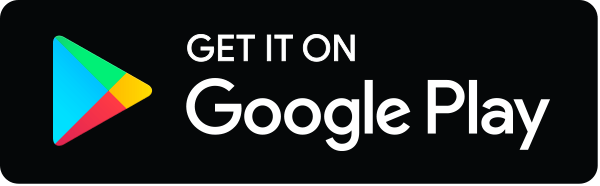