KEY POINTS
Proper management of fluid and electrolytes facilitates crucial homeostasis that allows cardiovascular perfusion, organ system function, and cellular mechanisms to respond to surgical illness.
Knowledge of the compartmentalization of body fluids forms the basis for understanding pathologic shifts in these fluid spaces in disease states. Although difficult to quantify, a deficiency in the functional extracellular fluid compartment often requires resuscitation with isotonic fluids in surgical and trauma patients.
Alterations in the concentration of serum sodium have profound effects on cellular function due to water shifts between the intracellular and extracellular spaces.
Different rates of compensation between respiratory and metabolic components of acid-base homeostasis require frequent laboratory reassessment during therapy.
Although active investigation continues, alternative resuscitation fluids have limited clinical utility, other than the correction of specific electrolyte abnormalities.
Most acute surgical illnesses are accompanied by some degree of volume loss or redistribution. Consequently, isotonic fluid administration is the most common initial intravenous fluid strategy, while attention is being given to alterations in concentration and composition.
Some surgical patients with neurologic illness, malnutrition, acute renal failure, or cancer require special attention to well-defined, disease-specific abnormalities in fluid and electrolyte status.
INTRODUCTION
Fluid and electrolyte management is paramount to the care of the surgical patient. Changes in both fluid volume and electrolyte composition occur preoperatively, intraoperatively, and postoperatively, as well as in response to trauma and sepsis. The sections that follow review the normal anatomy of body fluids, electrolyte composition and concentration abnormalities and treatments, common metabolic derangements, and alternative resuscitative fluids. These concepts are then discussed in relationship to management of specific surgical patients and their commonly encountered fluid and electrolyte abnormalities.
BODY FLUIDS
Water constitutes approximately 50% to 60% of total body weight. The relationship between total body weight and total body water (TBW) is relatively constant for an individual and is primarily a reflection of body fat. Lean tissues such as muscle and solid organs have higher water content than fat and bone. As a result, young, lean males have a higher proportion of body weight as water than elderly or obese individuals. Deuterium oxide and tritiated water have been used in clinical research to measure TBW by indicator dilution methods. In an average young adult male, TBW accounts for 60% of total body weight, whereas in an average young adult female, it is 50%.1 The lower percentage of TBW in females correlates with a higher percentage of adipose tissue and lower percentage of muscle mass in most. Estimates of percentage of TBW should be adjusted downward approximately 10% to 20% for obese individuals and upward by 10% for malnourished individuals. The highest percentage of TBW is found in newborns, with approximately 80% of their total body weight comprised of water. This decreases to approximately 65% by 1 year of age and thereafter remains fairly constant.
TBW is divided into three functional fluid compartments: plasma, extravascular interstitial fluid, and intracellular fluid (Fig. 3-1). The extracellular fluids (ECF), plasma and interstitial fluid, together compose about one third of the TBW, and the intracellular compartment composes the remaining two thirds. The extracellular water composes 20% of the total body weight and is divided between plasma (5% of body weight) and interstitial fluid (15% of body weight). Intracellular water makes up approximately 40% of an individual’s total body weight, with the largest proportion in the skeletal muscle mass. ECF is measured using indicator dilution methods. The distribution volumes of NaBr and radioactive sulfate have been used to measure ECF in clinical research. Measurement of the intracellular compartment is then determined indirectly by subtracting the measured ECF from the simultaneous TBW measurement.
The normal chemical composition of the body fluid compartments is shown in Fig. 3-2. The ECF compartment is balanced between sodium, the principal cation, and chloride and bicarbonate, the principal anions. The intracellular fluid compartment is composed primarily of the cations potassium and magnesium, and the anions phosphate and sulfate, and proteins. The concentration gradient between compartments is maintained by adenosine triphosphate–driven sodium-potassium pumps located with in the cell membranes. The composition of the plasma and interstitial fluid differs only slightly in ionic composition. The slightly higher protein content (organic anions) in plasma results in a higher plasma cation composition relative to the interstitial fluid, as explained by the Gibbs-Donnan equilibrium equation. Proteins add to the osmolality of the plasma and contribute to the balance of forces that determine fluid balance across the capillary endothelium. Although the movement of ions and proteins between the various fluid compartments is restricted, water is freely diffusible. Water is distributed evenly throughout all fluid compartments of the body so that a given volume of water increases the volume of any one compartment relatively little. Sodium, however, is confined to the ECF compartment, and because of its osmotic and electrical properties, it remains associated with water. Therefore, sodium-containing fluids are distributed throughout the ECF and add to the volume of both the intravascular and interstitial spaces. Although the administration of sodium-containing fluids expands the intravascular volume, it also expands the interstitial space by approximately three times as much as the plasma.
The physiologic activity of electrolytes in solution depends on the number of particles per unit volume (millimoles per liter, or mmol/L), the number of electric charges per unit volume (milliequivalents per liter, or mEq/L), and the number of osmotically active ions per unit volume (milliosmoles per liter, or mOsm/L). The concentration of electrolytes usually is expressed in terms of the chemical combining activity, or equivalents. An equivalent of an ion is its atomic weight expressed in grams divided by the valence:
Equivalent = atomic weight (g)/valence
For univalent ions such as sodium, 1 mEq is the same as 1 mmol. For divalent ions such as magnesium, 1 mmol equals 2 mEq. The number of milliequivalents of cations must be balanced by the same number of milliequivalents of anions. However, the expression of molar equivalents alone does not allow a physiologic comparison of solutes in a solution.
The movement of water across a cell membrane depends primarily on osmosis. To achieve osmotic equilibrium, water moves across a semipermeable membrane to equalize the concentration on both sides. This movement is determined by the concentration of the solutes on each side of the membrane. Osmotic pressure is measured in units of osmoles (osm) or milliosmoles (mOsm) that refer to the actual number of osmotically active particles. For example, 1 mmol of sodium chloride contributes to 2 mOsm (one from sodium and one from chloride). The principal determinants of osmolality are the concentrations of sodium, glucose, and urea (blood urea nitrogen, or BUN):
Calculated serum osmolality = 2 sodium + (glucose/18) + (BUN/2.8)
The osmolality of the intracellular and extracellular fluids is maintained between 290 and 310 mOsm in each compartment. Because cell membranes are permeable to water, any change in osmotic pressure in one compartment is accompanied by a redistribution of water until the effective osmotic pressure between compartments is equal. For example, if the ECF concentration of sodium increases, there will be a net movement of water from the intracellular to the extracellular compartment. Conversely, if the ECF concentration of sodium decreases, water will move into the cells. Although the intracellular fluid shares in losses that involve a change in concentration or composition of the ECF, an isotonic change in volume in either one of the compartments is not accompanied by the net movement of water as long as the ionic concentration remains the same. For practical clinical purposes, most significant gains and losses of body fluid are directly from the extracellular compartment.
BODY FLUID CHANGES
The healthy person consumes an average of 2000 mL of water per day, approximately 75% from oral intake and the rest extracted from solid foods. Daily water losses include 800 to 1200 mL in urine, 250 mL in stool, and 600 mL in insensible losses. Insensible losses of water occur through both the skin (75%) and lungs (25%) and can be increased by such factors as fever, hypermetabolism, and hyperventilation. Sensible water losses such as sweating or pathologic loss of gastrointestinal (GI) fluids vary widely, but these include the loss of electrolytes as well as water (Table 3-1). To clear the products of metabolism, the kidneys must excrete a minimum of 500 to 800 mL of urine per day, regardless of the amount of oral intake.
ROUTES | AVERAGE DAILY VOLUME (mL) | MINIMAL (mL) | MAXIMAL (mL) |
---|---|---|---|
H2O gain: | |||
Sensible: | |||
Oral fluids | 800–1500 | 0 | 1500/h |
Solid foods | 500–700 | 0 | 1500 |
Insensible: | |||
Water of oxidation | 250 | 125 | 800 |
Water of solution | 0 | 0 | 500 |
H2O loss: | |||
Sensible: | |||
Urine | 800–1500 | 300 | 1400/h |
Intestinal | 0–250 | 0 | 2500/h |
Sweat | 0 | 0 | 4000/h |
Insensible: | |||
Lungs and skin | 600 | 600 | 1500 |
The typical individual consumes 3 to 5 g of dietary salt per day, with the balance maintained by the kidneys. With hyponatremia or hypovolemia, sodium excretion can be reduced to as little as 1 mEq/d or maximized to as much as 5000 mEq/d to achieve balance except in people with salt-wasting kidneys. Sweat is hypotonic, and sweating usually results in only a small sodium loss. GI losses are isotonic to slightly hypotonic and contribute little to net gain or loss of free water when measured and appropriately replaced by isotonic salt solutions.
Disorders in fluid balance may be classified into three general categories: disturbances in (a) volume, (b) concentration, and (c) composition. Although each of these may occur simultaneously, each is a separate entity with unique mechanisms demanding individual correction. Isotonic gain or loss of salt solution results in extracellular volume changes, with little impact on intracellular fluid volume. If free water is added or lost from the ECF, water will pass between the ECF and intracellular fluid until solute concentration or osmolarity is equalized between the compartments. Unlike with sodium, the concentration of most other ions in the ECF can be altered without significant change in the total number of osmotically active particles, producing only a compositional change. For instance, doubling the serum potassium concentration will profoundly alter myocardial function without significantly altering volume or concentration of the fluid spaces.
Extracellular volume deficit is the most common fluid disorder in surgical patients and can be either acute or chronic. Acute volume deficit is associated with cardiovascular and central nervous system signs, whereas chronic deficits display tissue signs, such as a decrease in skin turgor and sunken eyes, in addition to cardiovascular and central nervous system signs (Table 3-2).Laboratory examination may reveal an elevated blood urea nitrogen level if the deficit is severe enough to reduce glomerular filtration and hemoconcentration. Urine osmolality usually will be higher than serum osmolality, and urine sodium will be low, typically <20 mEq/L. Serum sodium concentration does not necessarily reflect volume status and therefore may be high, normal, or low when a volume deficit is present. The most common cause of volume deficit in surgical patients is a loss of GI fluids (Table 3-3) from nasogastric suction, vomiting, diarrhea, or enterocutaneous fistula. In addition, sequestration secondary to soft tissue injuries, burns, and intra-abdominal processes such as peritonitis, obstruction, or prolonged surgery can also lead to massive volume deficits.
SYSTEM | VOLUME DEFICIT | VOLUME EXCESS |
---|---|---|
Generalized | Weight loss | Weight gain |
Decreased skin turgor | Peripheral edema | |
Cardiac | Tachycardia | Increased cardiac output |
Orthostasis/hypotension | Increased central venous pressure | |
Collapsed neck veins | Distended neck veins | |
Murmur | ||
Renal | Oliguria | — |
Azotemia | ||
GI | Ileus | Bowel edema |
Pulmonary | — | Pulmonary edema |
TYPE OF SECRETION | VOLUME (mL/24 h) | Na (mEq/L) | K (mEq/L) | Cl (mEq/L) | HCO3− (mEq/L) |
---|---|---|---|---|---|
Stomach | 1000–2000 | 60–90 | 10–30 | 100–130 | 0 |
Small intestine | 2000–3000 | 120–140 | 5–10 | 90–120 | 30–40 |
Colon | — | 60 | 30 | 40 | 0 |
Pancreas | 600–800 | 135–145 | 5–10 | 70–90 | 95–115 |
Bile | 300–800 | 135–145 | 5–10 | 90–110 | 30–40 |
Extracellular volume excess may be iatrogenic or secondary to renal dysfunction, congestive heart failure, or cirrhosis. Both plasma and interstitial volumes usually are increased. Symptoms are primarily pulmonary and cardiovascular (see Table 3-2). In fit patients, edema and hyperdynamic circulation are common and well tolerated. However, the elderly and patients with cardiac disease may quickly develop congestive heart failure and pulmonary edema in response to only a moderate volume excess.
Volume changes are sensed by both osmoreceptors and baroreceptors. Osmoreceptors are specialized sensors that detect even small changes in fluid osmolality and drive changes in thirst and diuresis through the kidneys.2 For example, when plasma osmolality is increased, thirst is stimulated and water consumption increases, although the exact cell mechanism is not known.3 Additionally, the hypothalamus is stimulated to secrete vasopressin, which increases water reabsorption in the kidneys. Together, these two mechanisms return the plasma osmolality to normal. Baroreceptors also modulate volume in response to changes in pressure and circulating volume through specialized pressure sensors located in the aortic arch and carotid sinuses.4 Baroreceptor responses are both neural, through sympathetic and parasympathetic pathways, and hormonal, through substances including renin-angiotensin, aldosterone, atrial natriuretic peptide, and renal prostaglandins. The net result of alterations in renal sodium excretion and free water reabsorption is restoration of volume to the normal state.
Changes in serum sodium concentration are inversely proportional to TBW. Therefore, abnormalities in TBW are reflected by abnormalities in serum sodium levels.
A low serum sodium level occurs when there is an excess of extracellular water relative to sodium. Extracellular volume can be high, normal, or low (Fig. 3-3). In most cases of hyponatremia, sodium concentration is decreased as a consequence of either sodium depletion or dilution.5 Dilutional hyponatremia frequently results from excess extracellular water and therefore is associated with a high extracellular volume status. Excessive oral water intake or iatrogenic intravenous (IV) excess free water administration can cause hyponatremia. Postoperative patients are particularly prone to increased secretion of antidiuretic hormone (ADH), which increases reabsorption of free water from the kidneys with subsequent volume expansion and hyponatremia. This is usually self-limiting in that both hyponatremia and volume expansion decrease ADH secretion. Additionally, a number of drugs can cause water retention and subsequent hyponatremia, such as the antipsychotics and tricyclic antidepressants as well as angiotensin-converting enzyme inhibitors. The elderly are particularly susceptible to drug-induced hyponatremia. Physical signs of volume overload usually are absent, and laboratory evaluation reveals hemodilution. Depletional causes of hyponatremia are associated with either a decreased intake or increased loss of sodium-containing fluids. A concomitant ECF volume deficit is common. Causes include decreased sodium intake, such as consumption of a low-sodium diet or use of enteral feeds, which are typically low in sodium; GI losses from vomiting, prolonged nasogastric suctioning, or diarrhea; and renal losses due to diuretic use or primary renal disease.
Hyponatremia also can be seen with an excess of solute relative to free water, such as with untreated hyperglycemia or mannitol administration. Glucose exerts an osmotic force in the extracellular compartment, causing a shift of water from the intracellular to the extracellular space. Hyponatremia therefore can be seen when the effective osmotic pressure of the extracellular compartment is normal or even high. When hyponatremia in the presence of hyperglycemia is being evaluated, the corrected sodium concentration should be calculated as follows:
For every 100-mg/dL increment in plasma glucose above normal, the plasma sodium should decrease by 1.6 mEq/L
Lastly, extreme elevations in plasma lipids and proteins can cause pseudohyponatremia, because there is no true decrease in extracellular sodium relative to water.
Signs and symptoms of hyponatremia (Table 3-4) are dependent on the degree of hyponatremia and the rapidity with which it occurred. Clinical manifestations primarily have a central nervous system origin and are related to cellular water intoxication and associated increases in intracranial pressure. Oliguric renal failure also can be a rapid complication in the setting of severe hyponatremia.
BODY SYSTEM | HYPONATREMIA |
---|---|
Central nervous system | Headache, confusion, hyperactive or hypoactive deep tendon reflexes, seizures, coma, increased intracranial pressure |
Musculoskeletal | Weakness, fatigue, muscle cramps/twitching |
GI | Anorexia, nausea, vomiting, watery diarrhea |
Cardiovascular | Hypertension and bradycardia if intracranial pressure increases significantly |
Tissue | Lacrimation, salivation |
Renal | Oliguria |
Body System | Hypernatremia |
Central nervous system | Restlessness, lethargy, ataxia, irritability, tonic spasms, delirium, seizures, coma |
Musculoskeletal | Weakness |
Cardiovascular | Tachycardia, hypotension, syncope |
Tissue | Dry sticky mucous membranes, red swollen tongue, decreased saliva and tears |
Renal | Oliguria |
Metabolic | Fever |
A systematic review of the etiology of hyponatremia should reveal its cause in a given instance. Hyperosmolar causes, including hyperglycemia or mannitol infusion and pseudohyponatremia, should be easily excluded. Next, depletional versus dilutional causes of hyponatremia are evaluated. In the absence of renal disease, depletion is associated with low urine sodium levels (<20 mEq/L), whereas renal sodium wasting shows high urine sodium levels (>20 mEq/L). Dilutional causes of hyponatremia usually are associated with hypervolemic circulation. A normal volume status in the setting of hyponatremia should prompt an evaluation for a syndrome of inappropriate secretion of ADH.
Hypernatremia results from either a loss of free water or a gain of sodium in excess of water. Like hyponatremia, it can be associated with an increased, normal, or decreased extracellular volume (see Fig. 3-3). Hypervolemic hypernatremia usually is caused either by iatrogenic administration of sodium-containing fluids, including sodium bicarbonate, or mineralocorticoid excess as seen in hyperaldosteronism, Cushing’s syndrome, and congenital adrenal hyperplasia. Urine sodium concentration is typically >20 mEq/L, and urine osmolarity is >300 mOsm/L. Normovolemic hypernatremia can result from renal causes, including diabetes insipidus, diuretic use, and renal disease, or from nonrenal water loss from the GI tract or skin, although the same conditions can result in hypovolemic hypernatremia. When hypovolemia is present, the urine sodium concentration is <20 mEq/L and urine osmolarity is <300 to 400 mOsm/L. Nonrenal water loss can occur secondary to relatively isotonic GI fluid losses such as that caused by diarrhea, to hypotonic skin fluid losses such as loss due to fever, or to losses via tracheotomies during hyperventilation. Additionally, thyrotoxicosis can cause water loss, as can the use of hypertonic glucose solutions for peritoneal dialysis. With nonrenal water loss, the urine sodium concentration is <15 mEq/L and the urine osmolarity is >400 mOsm/L.
Symptomatic hypernatremia usually occurs only in patients with impaired thirst or restricted access to fluid, because thirst will result in increased water intake. Symptoms are rare until the serum sodium concentration exceeds 160 mEq/L but, once present, are associated with significant morbidity and mortality. Because symptoms are related to hyperosmolarity, central nervous system effects predominate (see Table 3-4). Water shifts from the intracellular to the extracellular space in response to a hyperosmolar extracellular space, which results in cellular dehydration. This can put traction on the cerebral vessels and lead to subarachnoid hemorrhage. Central nervous system symptoms can range from restlessness and irritability to seizures, coma, and death. The classic signs of hypovolemic hypernatremia, (tachycardia, orthostasis, and hypotension) may be present, as well as the unique findings of dry, sticky mucous membranes.
The average dietary intake of potassium is approximately 50 to 100 mEq/d, which in the absence of hypokalemia is excreted primarily in the urine. Extracellular potassium is maintained within a narrow range, principally by renal excretion of potassium, which can range from 10 to 700 mEq/d. Although only 2% of the total body potassium (4.5 mEq/L × 14 L = 63 mEq) is located within the extracellular compartment, this small amount is critical to cardiac and neuromuscular function; thus, even minor changes can have major effects on cardiac activity. The intracellular and extracellular distribution of potassium is influenced by a number of factors, including surgical stress, injury, acidosis, and tissue catabolism.
Hyperkalemia is defined as a serum potassium concentration above the normal range of 3.5 to 5.0 mEq/L. It is caused by excessive potassium intake, increased release of potassium from cells, or impaired potassium excretion by the kidneys (Table 3-5).6 Increased intake can be either from oral or IV supplementation, or from red cell lysis after transfusion. Hemolysis, rhabdomyolysis, and crush injuries can disrupt cell membranes and release intracellular potassium into the ECF. Acidosis and a rapid rise in extracellular osmolality from hyperglycemia or IV mannitol can raise serum potassium levels by causing a shift of potassium ions to the extracellular compartment.7 Because 98% of total body potassium is in the intracellular fluid compartment, even small shifts of intracellular potassium out of the intracellular fluid compartment can lead to a significant rise in extracellular potassium. A number of medications can contribute to hyperkalemia, particularly in the presence of renal insufficiency, including potassium-sparing diuretics, angiotensin-converting enzyme inhibitors, and nonsteroidal anti-inflammatory drugs (NSAIDs). Spironolactone and angiotensin-converting enzyme inhibitors interfere with aldosterone activity, inhibiting the normal renal mechanism of potassium excretion. Acute and chronic renal insufficiency also impairs potassium excretion.
Hyperkalemia Increased intake Potassium supplementation Blood transfusions Endogenous load/destruction: hemolysis, rhabdomyolysis, crush injury, gastrointestinal hemorrhage Increased release Acidosis Rapid rise of extracellular osmolality (hyperglycemia or mannitol) Impaired excretion Potassium-sparing diuretics Renal insufficiency/failure Hypokalemia Inadequate intake Dietary, potassium-free intravenous fluids, potassium-deficient TPN Excessive potassium excretion Hyperaldosteronism Medications GI losses Direct loss of potassium from GI fluid (diarrhea) Renal loss of potassium (to conserve sodium in response to gastric losses) |
Symptoms of hyperkalemia are primarily GI, neuromuscular, and cardiovascular (Table 3-6). GI symptoms include nausea, vomiting, intestinal colic, and diarrhea. Neuromuscular symptoms range from weakness to ascending paralysis to respiratory failure. Early cardiovascular signs may be apparent from electrocardiogram (ECG) changes and eventually lead to hemodynamic symptoms of arrhythmia and cardiac arrest. ECG changes that may be seen with hyperkalemia include high peaked T waves (early), widened QRS complex, flattened P wave, prolonged PR interval (first-degree block), sine wave formation, and ventricular fibrillation.
INCREASED SERUM LEVELS | |||
---|---|---|---|
SYSTEM | POTASSIUM | MAGNESIUM | CALCIUM |
GI | Nausea/vomiting, colic, diarrhea | Nausea/vomiting | Anorexia, nausea/vomiting, abdominal pain |
Neuromuscular | Weakness, paralysis, respiratory failure | Weakness, lethargy, decreased reflexes | Weakness, confusion, coma, bone pain |
Cardiovascular | Arrhythmia, arrest | Hypotension, arrest | Hypertension, arrhythmia, polyuria |
Renal | — | — | Polydipsia |
DECREASED SERUM LEVELS | |||
SYSTEM | POTASSIUM | MAGNESIUM | CALCIUM |
GI | Ileus, constipation | — | — |
Neuromuscular | Decreased reflexes, fatigue, weakness, paralysis | Hyperactive reflexes, muscle tremors, tetany, seizures | Hyperactive reflexes, paresthesias, carpopedal spasm, seizures |
Cardiovascular | Arrest | Arrhythmia | Heart failure |
Hypokalemia is much more common than hyperkalemia in the surgical patient. It may be caused by inadequate potassium intake; excessive renal potassium excretion; potassium loss in pathologic GI secretions, such as with diarrhea, fistulas, vomiting, or high nasogastric output; or intracellular shifts from metabolic alkalosis or insulin therapy (see Table 3-5). The change in potassium associated with alkalosis can be calculated by the following formula:
Potassium decreases by 0.3 mEq/L for every 0.1 increase in pH above normal.
Additionally, drugs such as amphotericin, aminoglycosides, cisplatin, and ifosfamide that induce magnesium depletion cause renal potassium wastage.8,9 In cases in which potassium deficiency is due to magnesium depletion,10 potassium repletion is difficult unless hypomagnesemia is first corrected.
The symptoms of hypokalemia (see Table 3-6), like those of hyperkalemia, are primarily related to failure of normal contractility of GI smooth muscle, skeletal muscle, and cardiac muscle. Findings may include ileus, constipation, weakness, fatigue, diminished tendon reflexes, paralysis, and cardiac arrest. In the setting of ECF depletion, symptoms may be masked initially and then worsened by further dilution during volume repletion. ECG changes suggestive of hypokalemia include U waves, T-wave flattening, ST-segment changes, and arrhythmias (with digitalis therapy).
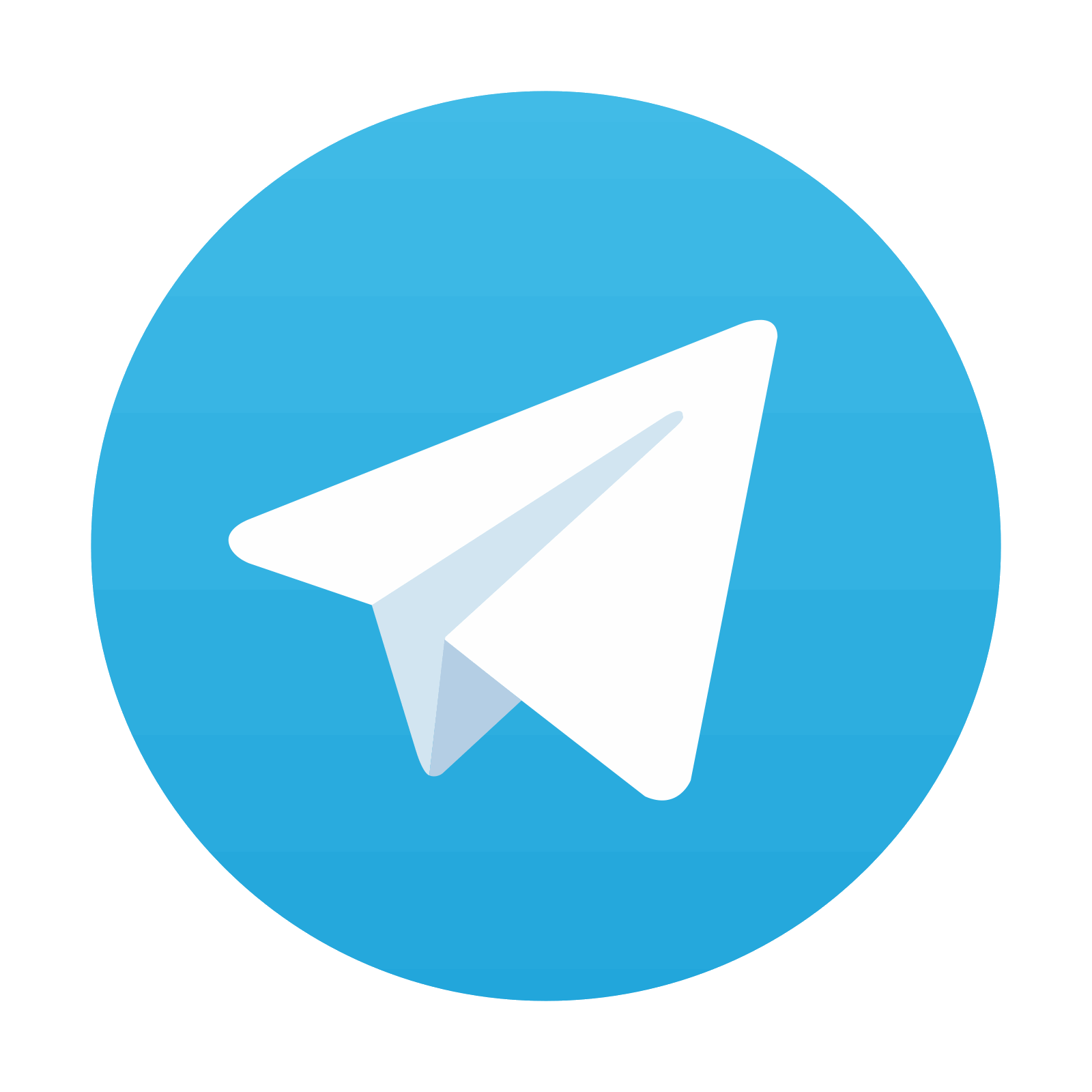
Stay updated, free articles. Join our Telegram channel
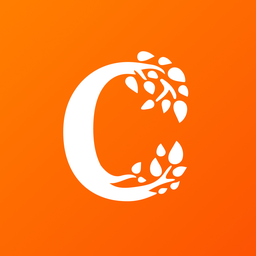
Full access? Get Clinical Tree
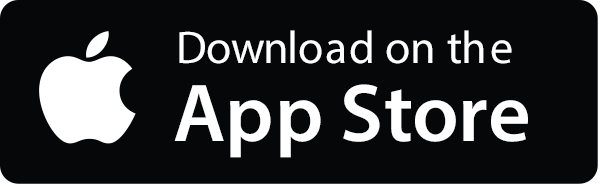
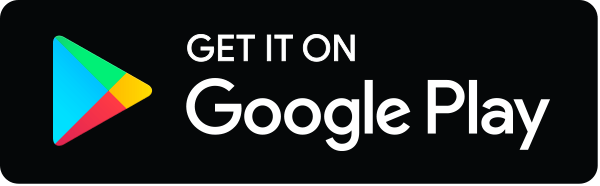