Objectives
- Identify the normal range of plasma glucose concentrations and the hormonal regulation of its metabolism, storage, and mobilization.
- Identify the specific roles of insulin, glucagon, glucocorticoids, catecholamines, growth hormone, and thyroid hormone in the regulation of energy substrate utilization, storage, and mobilization.
- Describe the hormonal regulation of energy substrate metabolism during the fed and fasted states and understand the consequences of its dysregulation.
- Identify the mechanisms involved in the maintenance of long-term energy balance.
- Identify the normal range of dietary sodium intake, its body distribution, and routes of excretion. Explain the roles of antidiuretic hormone, aldosterone, angiotensin, and atrial natriuretic hormone in the regulation of sodium balance.
- Identify the normal range of dietary potassium intake, its body distribution, and routes of excretion. Explain the hormonal regulation of plasma potassium concentration, distribution, and balance in the acute and chronic settings.
- Identify the normal range of dietary calcium intake, its body distribution, and routes of excretion. Explain the hormonal regulation of plasma calcium concentration through bone resorption, renal excretion, and intestinal absorption.
- Identify the normal range of dietary phosphate intake, its body distribution, and routes of excretion. Explain the hormonal regulation of plasma phosphate concentration through exchange with bone, renal excretion, and dietary intake and absorption.
Endocrine Integration of Energy and Electrolyte Balance: Introduction
In the first chapter, several of the key functions of the endocrine system that maintain homeostasis were outlined. Subsequent chapters described the specific physiologic effects of individual hormones, the mechanisms that regulate their production and release, and the consequences of isolated excess or deficiency. The presentation of this material would not be complete without an attempt to integrate some of these actions into the overall regulation of specific functions. Although a complete description of the integrative control of physiologic function is beyond the scope of this book, this chapter integrates many of the concepts already presented. It describes how the different arms of the neuroendocrine system interact to regulate and maintain basic functions, which include energy substrate balance, blood volume and blood pressure, and preservation of bone mineral density (BMD). Finally, it presents an integrated discussion of the neuroendocrine mechanisms involved in mediating the stress response.
Neuroendocrine Regulation of Energy Storage, Mobilization, and Utilization
Two distinct phases directly related to the ingestion of a meal alternate throughout the day in the regulation of energy metabolism. The fed state reflects overall anabolic metabolism, during which energy is stored in the form of energy-rich compounds (adenosine triphosphate [ATP], phosphocreatinine), glycogen, fat, and proteins. The fasted or catabolic phase is the period during which endogenous energy sources are utilized.
The anabolic and catabolic phases alternate to preserve adequate glucose supply to the brain as well as sufficient energy to maintain body functions, such as thermoregulation (maintaining a constant core temperature), food digestion, and physical activity. The 2 hormones at the core of maintaining this balance are insulin and glucagon (see Chapter 7); in particular, their ratio plays a critical role in the dynamic regulation of substrate metabolism (summarized in Table 10–1). However, several other established and newly discovered hormones participate in the regulation of energy metabolism to different extents, according to age, sex, nutritional state, and metabolic demands of the individual.
Anabolic ↑ I : G | Metabolic process | Catabolic ↓ I : G |
---|---|---|
↑ | Glycogen synthesis (liver and muscle) | ↓ |
↓ | Glycogen breakdown | ↑ |
↓ | Gluconeogenesis | ↑ |
↑ | Triglyceride synthesis (hepatocytes and adipose tissue) | ↓ |
↑ | Muscle protein synthesis | ↓ |
↑ | Lipogenesis and triglyceride formation | ↓ |
↓ | Lipolysis | ↑ |
↓ | Free fatty acid oxidation | ↑ |
↓ | Ketone body formation | ↑ |
↓ | Muscle proteolysis | ↑ |
The autonomic nervous system interacts with the endocrine system in the modulation of glucose and fat metabolism. Hence, the system is in fact under neuroendocrine regulation. The autonomic nervous system exerts its effects both directly and indirectly. For example, activation of the sympathetic nervous system through norepinephrine release directly stimulates skeletal muscle glycogenolysis and hepatic glucose output. The indirect effects of the autonomic nervous system are exemplified by sympathetic activation of the adrenal medulla (see Chapter 6), stimulating the release of epinephrine. Epinephrine stimulates the pancreatic release of glucagon and suppresses the release of insulin, resulting in an increase in the glucagon to insulin ratio and an overall increase in hepatic glucose production.
To simplify the discussion of the neuroendocrine regulation of substrate metabolism, a brief summary of overall substrate regulation and the principal hormones involved will be presented as they pertain to the fed (anabolic) and fasted (catabolic) states.
Blood glucose regulation occurs through interactions among hormonal, neural, and hepatic autoregulatory mechanisms. As described in detail in Chapter 7, the pancreatic hormones insulin and glucagon play central roles in the tight control of blood glucose concentrations. Following a meal (postprandial state), in response to the increase in pancreatic insulin release, glucose uptake is increased in muscle, fat, and the hepatosplanchnic bed; hepatic glucose output is suppressed; and glycogen synthesis is increased.
Glucose disposal by insulin-sensitive tissues is regulated initially by an increase in glucose transport and enzyme phosphorylation leading to the activation of glycogen synthase, phosphofructokinase, and pyruvate dehydrogenase (see Figure 7–5). The majority of insulin-stimulated glucose taken up is stored as glycogen. Hormonally induced changes in intracellular fructose 2,6-bisphosphate concentrations play a key role in muscle glycolytic flux and both glycolytic and gluconeogenic flux in the liver.
Most of the body’s energy reserve is stored in adipose tissue in the form of triglycerides. During periods of caloric excess or abundance, fat is stored in the form of triacylglycerol in the adipocytes. The principal hormone involved in lipogenesis is insulin, through activation of lipogenic and glycolytic enzymes. Opposing the effects of insulin are growth hormone (GH; discussed in Chapter 3) and leptin (described later), which inhibit lipogenesis (Figure 10–1). The balance between lipogenesis and lipolysis followed by fatty acid oxidation determines the overall accumulation of body fat.
Figure 10–1.
Hormonal factors controlling fat, protein, and carbohydrate (CHO) stores and balance. Adipose, protein, and carbohydrate (CHO in the form of glycogen) stores are the result of balanced synthesis and degradation under hormonal regulation by insulin (INS), growth hormone (GH), leptin, testosterone (T), epinephrine (Epi), thyroid hormone (TH), insulin-like growth factor 1 (IGF-1), and cortisol (Cort). Excess, deficiencies, or impaired regulation of adipose, protein, and carbohydrate stores have direct implications on health and disease as illustrated above.
The whole-body protein pool, as well as that of individual tissues, is determined by the balance between protein synthesis and degradation (see Figure 10–1). These in turn are principally regulated by interactions among hormonal, nutritional, neural, and inflammatory mediators. Hormonally, regulation of protein metabolism is predominantly under the influence of insulin, GH, and insulin-like growth factor-1 (IGF-1). During the fed state, insulin acts primarily to inhibit proteolysis, and GH stimulates protein synthesis. IGF-1 has antiproteolytic effects during the postabsorptive state that progress to stimulation of protein synthesis in the fed state or when amino acids are provided. GH and testosterone are of particular importance during growth and development, as well as during adulthood and senescence. Thyroid hormones are also required for normal growth and development. Thyroid hormones stimulate bone growth indirectly by increasing secretion of GH and IGF-1 and directly by activating gene transcription.
During the fasted postabsorptive state, catabolism of stored energy sources provides the energy required for bodily functions. The total amount of energy produced per unit of time by a given individual is referred to as the metabolic rate. The amount of energy expended by an awake, resting individual, measured 12–14 hours following the last meal, at normal (or thermoneutral) body temperature is called the basal metabolic rate (BMR). The BMR is the amount of energy required to maintain breathing, brain activity, enzymatic activity, and other functions without any physical movement of the individual. Any deviation from the basal condition, such as changes in body temperature (fever or hypothermia), the level of activity of the individual (exercise or sleeping), or time from the last meal (fed or fasted), will affect the metabolic rate. In addition, BMR can be directly affected by hormone action, particularly thyroid hormones, which increase body temperature and increase Na+/K+–adenosine triphosphatase (ATPase) activity, resulting in an increase in the BMR. In a healthy individual, BMR averages 2000 kcal/d. Hence, the recommended dietary allowance of calories is derived from the BMR, age, sex, and level of activity of any given individual. The BMR can be estimated clinically by measuring the amount of oxygen consumed with the use of indirect calorimetry.
In the resting postabsorptive state, release of glucose from the liver through glycogenolysis and gluconeogenesis is the key regulated process. During fasting, hepatic glucose production is increased and peripheral glucose utilization is inhibited. Initially, hepatic glucose output is derived from breakdown of hepatic glycogen stores (a maximum of 70–80 g in humans) through glycogenolysis. Following an overnight fast, glycogenolysis provides approximately 50% of the overall hepatic glucose output. As hepatic glycogen stores are depleted during a period of prolonged fasting (approximately 60 hours), the contribution of glycogenolysis to hepatic glucose output becomes negligible, with hepatic gluconeogenesis predominating. Glycogenolysis depends on the relative activities of glycogen synthase and phosphorylase, the latter being the more important (see Figure 7–5). Gluconeogenesis is regulated by the activities of fructose-1,6-diphosphatase, phosphoenolpyruvate carboxykinase, pyruvate kinase, and pyruvate dehydrogenase, and by the availability of the principal gluconeogenic precursors, lactate, glycerol, glutamine, and alanine.
A smaller, yet significant amount (approximately 25%) of systemic glucose production in the postabsorptive state is derived from renal gluconeogenesis. The proximal tubule cells produce glucose at a rate similar to that of glucose utilization by the renal medulla. Overall, the kidney is not a net producer of glucose. Thus, in the postabsorptive state, blood glucose homeostasis is the result of hormonal regulation of glycogenolysis, gluconeogenesis, and glucose uptake.
The amount of energy stored as triglycerides in adipose tissue is substantial. For example, an adult with 15 kg of body fat has enough energy to support the whole body energy requirements (8.37 MJ; 2000 kcal) for about 2 months. After an overnight fast, most of the resting energy requirement is provided by oxidation of fatty acids derived from adipose tissue. Lipolysis in adipose tissue is mostly dependent on the concentrations of hormones (epinephrine stimulates lipolysis, and insulin inhibits lipolysis). During a period of acute energy deprivation or prolonged starvation, lipolysis mobilizes triglycerides, providing nonesterified fatty acids as energy substrates for tissues such as muscle, heart, and liver; and substrates for glucose (glycerol) and lipoprotein (free fatty acids) synthesis to the liver. Unlike most other tissues, the brain cannot utilize fatty acids for energy when blood glucose levels become compromised. In this case, ketone bodies (discussed in Chapter 7) provide the brain with an alternative source of energy, amounting to nearly two-thirds of the brain’s energy needs during periods of prolonged fasting and starvation.
The release of glycerol and free fatty acids from adipose tissue is under negative regulation by insulin and is stimulated primarily by catecholamines (see Chapter 7). During fasting, plasma insulin levels decrease and plasma GH and glucagon both increase. As the fasting period progresses, or more frequently during periods of acute glucose deficiency (insulin-induced hypoglycemia) or increased energy demand (as with strenuous exercise), catecholamines play an important role in the stimulation of lipolysis.
Unlike excess fat and glucose, which are stored as fat and glycogen in adipose tissue, liver, and muscle, there is no storage pool for body protein. Therefore, under catabolic conditions, essential proteins are degraded. Glucagon, cortisol, and epinephrine together favor muscle protein breakdown and hepatic amino acid uptake, some of which can be utilized for gluconeogenesis (see Figure 10–1). The effects of glucagon are predominantly mediated through increased hepatic uptake of amino acids. Epinephrine increases production of the gluconeogenic amino acid alanine by muscle and its uptake by the splanchnic bed. Prolonged changes in either the protein synthetic or degradative processes (or both) leading to loss of lean body mass have been shown to increase morbidity and mortality from several diseases, including cancer and acquired immunodeficiency syndrome (AIDS).
Glucagon plays a primary role in energy metabolism. This hormone primarily stimulates hepatic glycogenolysis, as well as gluconeogenesis resulting in an overall increase in hepatic glucose output.
GH and cortisol facilitate glucose production and limit glucose utilization, but neither of them plays a critical role in the acute counterregulatory response to a hypoglycemic episode. Their effects are not immediate (delayed for approximately 6 hours); thus, they are mostly involved in defense against prolonged hypoglycemia. Cortisol contributes to the regulation of gluconeogenic substrate supply through permissive effects on the lipolytic action of catecholamines and GH in adipose tissue and on the glycogenolytic action of catecholamines in skeletal muscle. In addition, it induces hepatic enzymatic gene expression required for enhanced gluconeogenic rates and exerts permissive effects on the stimulation of gluconeogenesis in the liver by glucagon and epinephrine.
Epinephrine stimulates hepatic glycogenolysis and hepatic and renal gluconeogenesis, largely by mobilizing gluconeogenic precursors including lactate, alanine, glutamine, and glycerol from adipose and muscle stores. Epinephrine also limits glucose utilization by insulin-sensitive tissues. Epinephrine helps increase hepatic glucose output and together with glucagon acts within minutes to increase plasma glucose concentrations.
The increased activation of the sympathetic nervous system and the associated release of epinephrine and norepinephrine suppress pancreatic insulin release and stimulate glucagon release leading to an increased glucagon to insulin ratio. Thus, stimulation of glycogenolysis and inhibition of glycogen synthesis by glucagon and epinephrine as well as the glucagon-stimulated hepatic gluconeogenesis proceed unopposed.
The contribution of the activation of the autonomic nervous system is easier understood when described in the context of acute and severe hypoglycemia. Because of the clear advantages of glycemic control in preventing organ injury in diabetic patients, tight regulation of plasma glucose levels is desired and recommended in this patient population. However, the most prevalent problem associated with tight glycemic control is the development of insulin-induced hypoglycemia. The decrease in plasma glucose concentrations (hypoglycemia) within and below the physiologic postabsorptive concentration range of approximately 70–110 mg/dL (3.9–6.1 mmol/L) triggers the activation of a counterregulatory neuroendocrine response. Acute insulin-induced hypoglycemia increases neuronal activity in the nucleus tractus solitarius and lateral hypothalamic glucosensors, resulting in increased sympathetic activity.
Enhanced sympathetic activity suppresses pancreatic insulin release and stimulates glucagon and epinephrine release, leading to increased hepatic glucose output. The additional release of GH and cortisol also contribute to the increase in hepatic glucose output and the suppression of tissue glucose uptake, partly through an increase in tissue fatty acid oxidation. As plasma glucose levels are restored, peripheral glucose sensors in the portal vein, small intestine, and liver decrease firing. This afferent signal is transmitted to the hypothalamus and to the nucleus solitarius in the medulla through the vagus nerve, conveying information on the prevailing peripheral glucose levels. The hypothalamus integrates these signals and initiates an appropriate response through the inhibition of hepatic and adrenal nerve activity, with consequently decreased release of adrenomedullary catecholamines, removing the inhibition on pancreatic insulin release and thus allowing hyperglycemia to induce pancreatic insulin secretion. Thus, in this system, glucose acts as a feedback signal contributing to integration of the neuroendocrine mechanisms that regulate its homeostasis.
The neuroendocrine response to exercise is targeted to provide for the increased energy demands of the exercising muscle and includes activation of the sympathetic nervous system, release of GH, activation of the hypothalamic-pituitary-adrenal axis with consequent release of catecholamines and cortisol, suppression of insulin release, and stimulation of glucagon release (Figure 10–2). This neuroendocrine response stimulates lipolysis, and hepatic and muscle glycogenolysis leading to an increase in free fatty acids, gluconeogenic substrate mobilization, and hepatic glucose output (because of an increase in both gluconeogenesis and glycogenolysis). Both hepatic and skeletal muscle glycogenolysis are stimulated by the increase in catecholamine release. However, because muscle lacks glucose-6-phosphatase, the glucose-6-phosphate produced by muscle glycogenolysis is either oxidized in muscle cells or released into the circulation as lactate. The increased lactate delivered to the liver is then used for hepatic gluconeogenesis. Hepatic glycogenolysis predominates during intense exercise, but gluconeogenesis contributes substantially to the increased hepatic glucose output during prolonged exercise as the liver glycogen stores decline and the supply of gluconeogenic precursors increases. As exercise intensity increases from mild to moderate and intense, energy substrate selection switches from lipid to carbohydrate dependence. GH and cortisol contribute only minimally to the exercise-induced increase in liver glucose output.
Figure 10–2.
Neuroendocrine response to exercise. The principal pathways activated by stress are the hypothalamic-pituitary-adrenal axis and sympathetic nervous system resulting in the increased release of corticotropin-releasing hormone (CRH), arginine vasopressin (AVP), catecholamines, endorphins, and growth hormone (GH). In the periphery, increased production and release of cortisol, glucagon and catecholamines, and suppressed release of insulin favor an overall catabolic response. Stimulation of hepatic glycogenolysis and gluconeogenesis, muscle glycogenolysis, and adipose tissue lipolysis ensure the production and mobilization of energy stores to sustain the enhanced metabolic demands of the individual. Reproductive and growth functions are inhibited, conserving energy to sustain fundamental processes that ensure survival. ACTH, adrenocorticotropic hormone; ATP, adenosine triphosphate; FA, fatty acid; FFA, free fatty acid; SNS, sympathetic nervous system.
Skeletal muscle is the main site of oxidation of fatty acids, and endogenous triacylglycerols represent an important source of energy both at rest and during low- and moderate-intensity exercise. During moderate-intensity exercise, lipolysis increases approximately 3-fold, mainly because of an increase in β-adrenergic stimulation, leading to an increased release of glycerol (used as a hepatic gluconeogenic substrate) and fatty acids into the circulation. The increase in adipose tissue and muscle blood flow facilitates the delivery of fatty acids to skeletal muscle for oxidation. In combination, the decrease in insulin and enhanced sympathetic stimulation, result in increased lipolysis and triacylglycerol oxidation; which serves as the main energy substrate for muscle. As the intensity of exercise increases, fat oxidation increases further until exercise intensities reach approximately 65% maximum oxygen consumption (VO2 max). After this point, the rate of fat oxidation declines, most likely because of reduced fatty acid delivery from adipose tissue to muscle.
The contribution of amino acid oxidation to total energy expenditure is negligible during short-term intense exercise and accounts for 3–6% of the total ATP supplied during prolonged exercise in humans. Although it is not quantitatively important regarding energy supply, the intermediary metabolism of several amino acids, notably glutamate, alanine, and the branched-chain amino acids, influences the availability of tricarboxylic acid cycle intermediates. Sustained dynamic exercise stimulates amino acid oxidation, chiefly of the branched-chain amino acids, and ammonia production in proportion to exercise intensity. If the exercise is intense enough, it causes a net loss of muscle protein (as a result of decreased protein synthesis, increased breakdown, or both); some of the amino acids are oxidized as energy, whereas the rest provide substrates for gluconeogenesis and possibly for acid-base regulation.
The neuroendocrine mechanisms involved in maintaining energy balance have been described earlier. Furthermore, the hormonal regulation of the 2 principal energy stores in the body—hepatic glycogen and adipose tissue triglycerides—has been outlined. The balanced transition from fed to fasted and the consumption of adequate energy commensurate with the level of physical activity of a given individual ensure that adequate energy reserves are available for short-term increases in metabolic demands, such as those described for exercise. An imbalance in either energy intake or expenditure leads to 1 of 2 extremes: loss of lean body mass or wasting syndrome resulting from an overall catabolic state, or obesity resulting from a combination of excess caloric intake and decreased physical activity. The salient features of these 2 conditions are described below.
When the fasted state is prolonged into a state of starvation, or under conditions of increased energy substrate demands, the contributions of the counterregulatory hormones cortisol, epinephrine, norepinephrine, and glucagon become evident, favoring overall catabolic effects. During such conditions, insulin effects are practically abolished. Because of the important anabolic effects of insulin, it follows that the metabolic responses that predominate are those that lead to catabolism of stored energy (glycogenolysis, lipolysis) and of lean tissues (muscle proteolysis), leading to loss of lean body mass, or wasting. It is important to note that energy metabolism can be pushed to a starvation-like state not only by discontinuation of food intake, in the classic sense of starvation, but also during conditions of stress, such as from a surgical intervention, cancer, severe infection, sepsis, burns, and traumatic injury. Under these conditions, additional factors such as inflammatory cytokines (eg, tumor necrosis factor [TNF], interleukins 1 and 6) interact with mediators of the neuroendocrine response. In addition, the production of GH, IGF-1, and the respective binding proteins becomes dysregulated, also contributing to the lack of anabolic processes and dominance of catabolic responses. Overall, the increased release of stress hormones and proinflammatory cytokines and the impairment in the release and action of insulin and growth factors, in association with altered release of androgens, lead to an overall catabolic response that affects the liver, adipose tissue, muscle, and, in extreme circumstances, visceral tissues. The deleterious impact of the loss of lean body mass on survival from disease underscores the relevance of understanding the hormonal mechanisms involved in the regulation of energy metabolism and their importance in the management of the critically ill patient.
Obesity is defined as a significant increase above the ideal weight (the weight that maximizes life expectancy). The increase in body mass index (BMI), an indicator of the adiposity or fatness that accompanies obesity, has become an important health problem for the developed world. Life expectancy is reduced when body mass index is significantly increased above the ideal level. Obesity is associated with an increased risk of diabetes, dyslipidemia, hypertension, heart disease, diabetes, and cancer. Approximately 20% of the US population is considered obese, according to the definition of the World Health Organization (Table 10–2).
BMI (kg/m2)* | Definition | WHO classification |
---|---|---|
<18.5 | Thin | |
18.5–24.9 | Healthy or normal | |
25.0–29.9 | Overweight | Grade 1 overweight |
30.0–39.9 | Obese | Grade 2 overweight |
=40.0 | Morbidly obese | Grade 3 overweight |
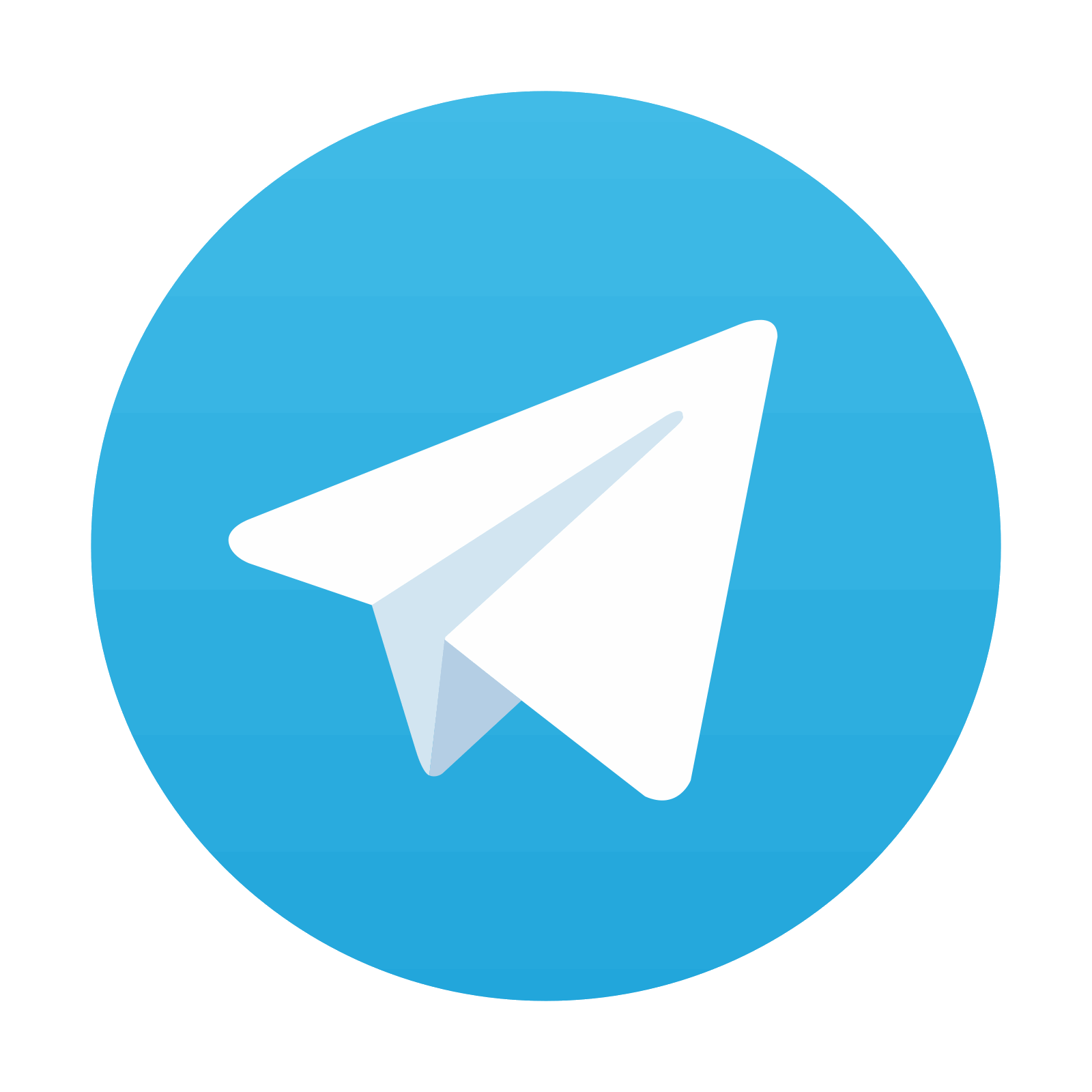
Stay updated, free articles. Join our Telegram channel
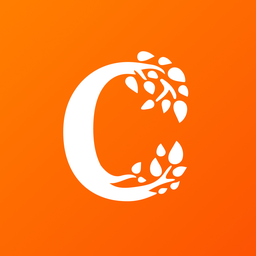
Full access? Get Clinical Tree
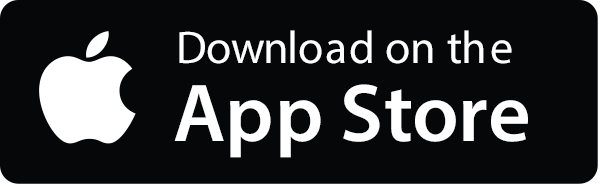
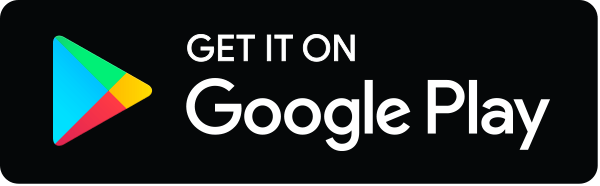