Disorders of Potassium Metabolism
Biff F. Palmer
Thomas D. DuBose Jr.
Introduction
Potassium plays a key role in maintaining cell function. All cells possess the ubiquitous Na+/K+ ATPase exchanger, which pumps Na+ out of, and K+ into, the cell. This leads to a K+ gradient across the cell membrane (K+in > K+out), which is partially responsible for maintaining the potential difference across the membrane. This potential difference is important to the function of all cells, but is especially important in excitable tissues such as nerve and muscle. For these reasons, the body has developed numerous mechanisms for defense of serum K+. Total body K+ is approximately 50 mEq/kg, which in a 70-kg person would be 3,500 mEq. The majority (98%) of this K+ is within cells with only 2% in the extracellular fluid. The normal concentration of K+ in the extracellular fluid is 3.5 to 5.3 mEq/L. Large deviations from these values are not compatible with life. Approximately 90% of the daily K+ intake is excreted in the urine, while 10% is excreted by the gastrointestinal tract.
In 2004, the Food and Nutrition Board of the Institute of Medicine established an adequate intake level for potassium of 4,700 mg/day. Data from NHANES 2007-2008 estimated the mean intake in the United States to be 2,290 mg/day for women and 3,026 mg/day for men, substantially lower than the recommended value (1). This relative “deficiency” of dietary K+ is even more noteworthy when one considers that the K+ intake of prehistoric man was estimated to be 15,000 mg/day, a value that exceeds the NHANES recommendations by a factor greater than 4 (2, 3). The mismatch between nutritional requirements encoded into the human genome during evolution and current intake is implicated in various chronic diseases to include hypertension, cardiovascular disease, osteoporosis, and nephrolithiasis. In this regard, dietary supplementation of K+ has favorable effects in reducing blood pressure, decreasing the risk of stroke, improving bone health, and reducing the risk of nephrolithiasis (4).
The normal kidney can maintain K+ homeostasis with great facility in the setting of high dietary intake. As an example, serum K+ levels are maintained in the normal range even when dietary K+ intake is increased to approximately 15 g daily for 20 days (5). In addition to the well-recognized role of aldosterone in renal K+ secretion, recent findings have identified the presence of an enteric K+ sensing mechanism that can initiate the renal secretory process upon K+ entry into the gastrointestinal tract. Additionally, the distal convoluted tubule (DCT) has been identified as a K+ sensor capable of initiating K+ exertion independent of mineralocorticoid activity. Before discussing clinical disorders of K+ homeostasis, these new insights into renal K+ handling will be reviewed.
Overview of Renal K+ Handling
K+ is freely filtered by the glomerulus and then avidly reabsorbed by the proximal tubule and thick ascending limb such that only a small amount reaches the distal nephron. Reabsorption in the proximal tubule is
primarily through the paracellular pathway and is in rough proportion to Na+ and water. The apical membrane Na+/K+/2Cl– cotransporter mediates transcellular K+ transport in the thick ascending limb of Henle. In the early DCT K+ secretion begins and progressively increases in magnitude into the cortical collecting duct. As recently reviewed, the secretory component of K+ handling is that which varies and is regulated according to physiologic needs (6).
primarily through the paracellular pathway and is in rough proportion to Na+ and water. The apical membrane Na+/K+/2Cl– cotransporter mediates transcellular K+ transport in the thick ascending limb of Henle. In the early DCT K+ secretion begins and progressively increases in magnitude into the cortical collecting duct. As recently reviewed, the secretory component of K+ handling is that which varies and is regulated according to physiologic needs (6).
The major K+-secretory mechanism in the distal nephron is electrogenic secretion through the ROMK (renal outer medullary K+) channel. A second channel (maxi-K+ or BK channel) also mediates K+ secretion under conditions of increased flow. In addition to stimulating maxi-K+ channels, tubular flow also augments electrogenic K+ secretion by diluting luminal K+ concentration and stimulating Na+ reabsorption through the epithelial Na+ channel (ENaC). In part, this stimulatory effect can be traced to a mechanosensitive property whereby shear stress increases the open probability of the ENaC channel (7).
The biomechanical characteristics of Na+ and K+ transport in the distal nephron are ideally suited to buffer any increase in extracellular K+ concentration following a K+-rich diet. A protein-enriched meal high in K+ content typical of early man would lead to an increase in glomerular filtration rate (GFR) and tubular flow (8). Increased flow through the distal nephron increases distal Na+ delivery and dilutes luminal K+ concentration both of which augment electrogenic K+ secretion through the ROMK channel. Along with flow-mediated activation of maxi-K+ channels, these events enhance renal K+ secretion thus providing a defense against development of hyperkalemia.
The renal response to a high-K+, low-Na+ diet has been studied in experimental rats (9, 10). Animals were fed a diet high in K+ and low in Na+ for several days and given exogenous deoxycorticosterone to ensure a steady state level of mineralocorticoid activity. Renal K+ handling was then examined following an acute KCl load. In the initial 2 hours following intraperitoneal injection of KCl, there was a large increase in renal K+ excretion primarily due to increased secretion into the colleting duct. This increased K+ secretory capacity is likely due to increased density of both ROMK and maxi-K+ channels which are both known to increase under conditions of high K+ intake (11). Over the next 4 hours renal K+ excretion continued to be high, but the kaliuresis was mostly due to high flow through the collecting duct. The timing of the two phases is important since the effect of high flow to simulate renal K+ secretion would be maximal only when K+ channels are maximally expressed.
Increased medullary recycling and accumulation of K+ in the interstitium of the kidney decreases Na+ reabsorption both in the thick ascending limb and proximal tubule thus providing a mechanism for high K+ intake to increase tubular flow (12, 13). Given the high capacity of the proximal tubule and thick ascending limb to reabsorb Na+, more recent studies have focused on how K+ intake modulates transport in the low capacity early DCT as a way to adjust tubular flow to K+ secretory sites. In the setting of a high-K+, low-Na+ diet, inhibiting transport in high-capacity upstream segments might lack the precision necessary to ensure delivery of Na+ appropriate to maximally stimulate K+ secretion and at the same time not be excessive predisposing to volume depletion.
The Distal Tubule as a K+ Sensor
The DCT is comprised of a proximal portion (DCT1) where salt transport is driven exclusively by the thiazide-sensitive NaCl cotransporter (NCC) (Fig. 5-1). In the distal part of the DCT (DCT2) electroneutral NaCl transport coexists with electrogenic Na+ and K+ transport pathways (14). Aldosterone sensitivity begins in the DCT2 and extends to the collecting duct. Changes in transport in the early DCT control the delivery of NaCl to the downstream connecting tubule and colleting duct where the epithelial sodium channel (ENaC) mediates electrogenic Na+ reabsorption and where K+ is secreted. In this regard, cells of the early DCT exert a substantial, albeit indirect, role in K+ secretion.
The low capacity nature of DCT1 and its location immediately upstream from the aldosterone-sensitive distal nephron (ASDN) makes this segment a more likely site for changes in dietary K+ intake to modulate Na+ transport and ensure downstream delivery of Na+ is precisely the amount needed to ensure maintenance of K+ homeostasis without causing unwanted effects on volume.
Increased dietary K+ intake leads to an inhibitory effect on Na+ transport in the DCT and does so through effects on members of the with no lysine (WNK) family of kinases (15). WNK1 and its shorter spliced variant KS-WNK1 (KS-kidney specific) are highly expressed in the DCT and connecting duct. KS-WNK1 functions as a physiologic antagonist to the actions of long WNK1. Changes in the ratio of KS-WNK1 and long WNK1 in response to dietary K+ contribute to the physiologic regulation of renal K+ excretion (16).
Under normal circumstances, long WNK1 prevents the ability of WNK4 (another member of the WNK family) to inhibit activity of the Na+/Cl– cotransporter in the DCT. Thus increased activity of long WNK1 leads to a net increase in NaCl reabsorption. Dietary K+ loading increases the abundance of KS-WNK1, which blocks the inhibitory effect of long WNK1 on WNK4. The net effect is inhibition of Na+/Cl– cotransport in the DCT and increased Na+ delivery to more distal parts of the tubule. The increase in KS-WNK1 in response to high K+ intake also antagonizes the effect of long WNK1 to stimulate endocytosis of ROMK. In addition, KS-WNK1 exerts a stimulatory effect on the ENaC. In total, increases in KS-WNK1 in response to dietary K+ loading facilitates K+ secretion through the combined effects of increased Na+ delivery through downregulation of Na+/Cl– cotransport in the early DCT increased electrogenic Na+ reabsorption via the ENaC and greater abundance of ROMK. These effects can occur independent of any change in mineralocorticoid activity suggesting an intrinsic sensing capability of this segment to changes in dietary K+.
Recent studies suggest extracellular K+ modulates the WNK axis by altering membrane voltage and changing intracellular chloride concentration (17, 18). An increased plasma K+ concentration, as with increased
dietary intake, would depolarize cells in the DCT1 resulting in increased intracellular Cl– concentration. This increase inhibits activity of WNK4 resulting in decreased activity of NCC. The unique sensitivity of WNK4 to Cl– is consistent with this model.
dietary intake, would depolarize cells in the DCT1 resulting in increased intracellular Cl– concentration. This increase inhibits activity of WNK4 resulting in decreased activity of NCC. The unique sensitivity of WNK4 to Cl– is consistent with this model.
High K+ intake also has a stimulatory effect on release of aldosterone at the level of the adrenal gland. Increased aldosterone complements the effect of KS-WNK1 in the DCT (19, 20). Aldosterone upregulates the serum- and glucocorticoid-dependent protein kinase (SGK1) that, in turn, phosphorylates WNK4. This modification prevents WNK4 from inhibiting ROMK and the ENaC (19, 21). SGK1 also increases ENaC expression and activity through effects on the ubiquitin-protein ligase Nedd4-2 (22). It should be emphasized that the absence of angiotensin II is a critical factor in the ability of high K+ intake to bring about the changes necessary to facilitate K+ secretion without excessive Na+ reabsorption, a phenomenon referred to as the aldosterone paradox.
K+-rich foods, such as fruit and vegetables, are also rich in precursors to bicarbonate ions. The alkali present in such a diet directly affects the determinants of K+ transport in the DCT so as to facilitate the renal excretion of the co-ingested K+ load (23, 24). For example, ENaC abundance is increased when luminal or basolateral HCO3– and pH is elevated. In addition, increased intracellular pH increases activity of ENaC, ROMK, and maxi-K+ channels. These effects of an alkaline pH provide an additional mechanism to facilitate K+ excretion following ingestion of such foods.
Enteric Sensing of K+ Intake
A number of enteric solute sensors capable of responding to dietary Na+/K+, and phosphate have been identified which signal the kidney to rapidly alter ion excretion or reabsorption (25, 26). In this regard, the ability to sense K+ within the gastrointestinal tract may have evolved as a way to rapidly initiate the kaliuretic response thereby facilitating maintenance of K+ homeostasis in the setting of high K+ intake. For example, the kaliuretic response to a dietary K+ load is greater when given as a meal compared to an intravenous infusion even in a setting where plasma K+ concentration is identical. Gastric delivery of K+ leads to nearly complete dephosphorylation of the Na+/Cl– cotransporter in the early DCT causing decreased activity of the transporter thus enhancing delivery of Na+ to the ASDN (27). The downstream shift in Na+ reabsorption from the DCT to the ENaC in the ASDN as well as increased maxi-K channel K+ secretion brought on by increased flow accounts for the increase in renal K+ excretion. This rapid natriuretic response to increases in dietary K+ intake is consistent with the blood pressure-lowering effect of K+-rich diets discussed further below. These data suggest splanchnic sensing of K+ can initiate the renal excretory response independent of change in plasma K+ concentration or mineralocorticoid activity.
Hypokalemia
APPROACH TO THE HYPOKALEMIC PATIENT
Hypokalemia is frequently encountered in clinical practice. Transient causes of hypokalemia are due to cell shift while sustained hypokalemia is either due to inadequate intake or excessive K+ loss. Hypokalemia resulting from excessive K+ loss can be due to renal or extrarenal losses. The clinical history, physical examination with particular emphasis on determination of volume status, and determination of the acid-base status will allow the cause of hypokalemia to be readily determined in most cases.
Assessment of renal K+ excretion allows one to determine whether hypokalemia is due to renal or extrarenal causes. Renal K+ handling can be assessed with a 24-hour urine collection or a spot urine determining the K+/creatinine ratio. A 24-hour urinary K+ of less than 15 mEq or a K+ (mmol)/creatinine (mmol) ratio less than 1 suggests an extrarenal cause of hypokalemia.
The main limitation to the use of a spot urine K+ is the influence of renal water handling on urine K+ concentration. Two patients with similar renal K+ excretion would have significantly different urine K+ concentrations depending on whether the urine was concentrated or dilute. The transtubular K+ gradient (TTKG) has been proposed as a useful tool to assess renal K+ handling and to overcome this variance.

The K+ concentration in the final urine will exceed the concentration at the beginning of the collecting duct as a result of water reabsorption along the length of the collecting duct. To account for this effect, the equation divides the urine K+ concentration by the ratio of the urine osmolality to serum osmolality. In an otherwise normal subject ingesting a typical western diet, the TTKG ranges from 8 to 9 and will increase to >11 with increased K+ intake. In a chronically hyperkalemic subject, a value <5 would indicate impaired renal K+ excretion either as a result of aldosterone deficiency or resistance. In patients with hypokalemia due to extrarenal K+ losses, the TTKG should fall to values <3.
It is worthwhile considering some of the assumptions made in calculating the TTKG. First, the calculation assumes there is no significant solute transport and only water reabsorption as fluid enters the medullary collecting duct. Any Na+ or urea reabsorption in this segment would tend to lower urine osmolality and cause the TTKG to overestimate the gradient for K+ secretion in the upstream collecting duct. Second, conditions should be optimal for K+ secretion at the time the TTKG is measured. In this regard, urine Na+ should be no less than 25 mEq/L to ensure Na+ delivery to the collecting duct is not rate limiting in K+ secretion. In addition, urine osmolality should be equal to and ideally greater than the plasma. A higher urine osmolality reflects increased vasopressin which is known to exert a stimulatory effect on K+ secretion in the collecting duct.
While the TTKG is of interest, in most settings, a spot urine K+ concentration and consideration of the clinical setting will be sufficient to determine the cause of K+ disturbances. Calculation of the TTKG may prove useful in those patients in which the cause of a dyskalemia continues to remain in doubt (28).
ETIOLOGY OF HYPOKALEMIA
Low K+ Intake
While the kidney can elaborate urine virtually free of Na+ in response to dietary Na+ restriction, the kidney can only reduce urinary K+ to approximately 15 mEq/day in response to a K+-free diet. As a result, extreme dietary restriction of K+ as might occur with a fad diet or eating disorder can conceivably lead to hypokalemia over time. An example of such dietary restriction might occur in patients with anorexia nervosa. Hypokalemia is of particular concern given its association with QT prolongation and ventricular arrhythmias (29). Such changes have been suggested to play a role in the increased risk of sudden death reported in these patients.
More commonly, dietary K+ restriction simply exacerbates the hypokalemia that is due to other causes. In a recent report, life-threatening hypokalemia developed in a patient with undiagnosed primary hyperaldosteronism after starting a low-carbohydrate, low-calorie diet (30). In addition to less dietary K+ intake, worsening hypokalemia can also be the result of increased renal K+ excretion in this setting. Low-carbohydrate diets are accompanied by a period of increased ketogenesis. The excretion of these acids by the kidney in the form of sodium salts results in increased distal delivery of Na+ in the setting of increased aldosterone. As a result, renal K+ wasting is exacerbated.
Cellular Redistribution
Since adjustments in renal K+ excretion can take several hours, changes in extracellular K+ concentration are initially buffered by movement of K+ into or out of the skeletal muscle. The two most important factors that regulate this movement under normal conditions are insulin and catecholamines. For example, following a meal, the postprandial release of insulin not only functions to regulate the serum glucose concentration but also functions to shift dietary K+ into cells until the kidney excretes the K+ load reestablishing normal total body K+ content. During exercise, the release of catecholamines through β2 stimulation limits the increase in extracellular K+ concentration that would otherwise occur as a result of the normal K+ release by the contracting muscle.
Pathologic stimulation of β2 receptors can result in symptomatic hypokalemia. For example, hypokalemic paralysis is a potential complication of the hyperadrenergic state that oftentimes accompanies alcohol withdrawal syndromes (31). Clenbuterol is a β2 adrenergic agonist with a rapid onset and long duration of action approved for limited use in veterinary medicine. The drug has been used illicitly as an alternative to anabolic steroids due to its effects to increase muscle mass. Hypokalemia as a result of clenbuterol toxicity has now been reported in users of heroin adulterated with clenbuterol (32).
Intracellular K+ also serves as a reservoir to limit the fall in extracellular K+ concentration that occurs under pathologic conditions in which there is loss of K+ from the body. An example of the efficiency of this buffering effect was previously reported in military recruits undergoing training in the summer (33). The trainees
were found to have K+ losses of >40 mEq/day in sweat alone. At the end of 11 days, subjects exhibited a total body K+ deficit of approximately 400 mEq and yet the serum K+ concentration was maintained near normal.
were found to have K+ losses of >40 mEq/day in sweat alone. At the end of 11 days, subjects exhibited a total body K+ deficit of approximately 400 mEq and yet the serum K+ concentration was maintained near normal.
Recent work has been devoted to better define the role of the skeletal muscle in regulating extracellular K+ concentration (34). In these studies, the movement of K+ into the skeletal muscle is indirectly determined by the use of a K+ clamp. With this technique, insulin is administered to rats at a constant rate. K+ is simultaneously infused at a rate designed to prevent any drop in plasma K+ concentration. Frequent measurements of plasma K+ values serve as a guide. The amount of K+ administered is presumed to be equal to the amount of K+ entering the intracellular compartment.
This model was used to study the effect of total body K+ depletion on insulin-stimulated K+ uptake. In rats deprived of K+ for 10 days, the plasma K+ concentration decreased from 4.2 to 2.9 mmol/L. Insulin-mediated K+ disappearance declined by more than 90% when compared to control values. This decrease in K+ uptake was accompanied by a >50% reduction in both the activity and expression of muscle Na+/K+ ATPase suggesting that decreased pump activity might account for the decrease in the effect of insulin. When measured after only 2 days of deprivation there was also a significant decline in insulin-mediated K+ uptake despite the fact that plasma K+ concentration had only decreased slightly (4.2-3.8 mmol/L). Interestingly, the expression and activity of the Na+/K+ ATPase was still normal suggesting the initial resistance to insulin-mediated K+ uptake is due to a mechanism other than decreased pump activity or expression. This decrease in muscle K+ uptake under conditions of K+ depletion may serve to limit an excessive decrease in extracellular K+ concentration that might otherwise occur under conditions in which insulin is stimulated. These changes would still allow skeletal muscle to buffer any decline in extracellular K+ concentration by donating some component of its intracellular stores.
Since chronic K+ depletion decreases skeletal muscle Na+/K+ ATPase expression and activity, one would expect that the ability to clear an acute K+ load under these conditions would be diminished and potentially result in dangerously high levels of plasma K+. This hypothesis was tested by administering intravenous KCl acutely to rats fed a K+-free diet for 2 weeks (35, 36). In contrast to what was predicted, total K+ clearance capacity was actually greater in the K+-depleted animals when compared to K+-replete controls. The skeletal muscle Na+/K+ ATPase pool size was decreased but the decrease was found to be specific to the α-2 isoform which is the major form found in the skeletal muscle. There was no change in the less abundant α-1 isoform. By contrast, cardiac Na+/K+ ATPase pool size increased in K+-deficient animals. Like the skeletal muscle, there was a decrease in the α-2 isoform but a significant increase in the α-1 isoform, which is the dominant form found in the heart. At baseline, the decrease in myocardial K+ content was considerably less compared to the skeletal muscle. Despite a smaller deficit of K+, the myocardial uptake of K+ during the intravenous infusion of K+ was of the same magnitude as skeletal muscle.
These findings indicate significant differences between skeletal and cardiac muscle in the response to K+ depletion. While the skeletal muscle readily relinquishes K+ to help minimize the drop in plasma K+ concentration, cardiac tissue K+ content remains relatively preserved. This difference can be attributed, at least in part, to Na+/K+ ATPase isoform differences in the response to K+ depletion. Cardiac muscle accumulates a considerable amount of K+ in the setting of an acute load. When expressed on a weight basis, the cardiac capacity for K+ uptake is comparable to that of the skeletal muscle under conditions of K+ depletion and may actually exceed the skeletal muscle under control conditions.
Potassium administration in patients with chronic hypokalemia may result in hyperkalemia. This response may be due to the chronic suppression of aldosterone elaboration by hypokalemia. Therefore, in summary, the rate of potassium replacement in the chronic hypokalemic patient should be relatively slow and monitored closely.
Hypokalemic periodic paralysis is a rare disorder that is characterized by muscle weakness or paralysis due to the sudden movement of K+ into cells. The attacks are precipitated by rest after exercise, stress, high-carbohydrate meals, and events accompanied by increased release of catecholamines or insulin. This disorder may be familial or acquired.
The acquired form of periodic paralysis typically develops in association with thyrotoxicosis (37). Thyrotoxic periodic paralysis is more commonly seen in Asians but has also been reported with higher frequency in Native Americans and Hispanics. While the incidence of thyrotoxicosis is typically more common in women, there is a male to female predominance that ranges from 17:1 to 70:1 in those who developed hypokalemic periodic paralysis. The typical patient is a young adult man age 20 to 40 who presents with weakness most commonly between the hours of 2100 and 0900 during the summer months. The attacks are precipitated by conditions characterized by increased release of catecholamines or insulin such as stress, high-carbohydrate meals, and exercise. With regard to exercise, the timing of attacks is typically in the initial rest period following
exertion. Oftentimes the attacks are heralded by muscle cramps and aches and many patients learn to avoid paralytic episodes by exercising the involved muscle groups. Hypophosphatemia and hypomagnesemia are also common during acute attacks and like K+ is the result of shifts into the intracellular compartment.
exertion. Oftentimes the attacks are heralded by muscle cramps and aches and many patients learn to avoid paralytic episodes by exercising the involved muscle groups. Hypophosphatemia and hypomagnesemia are also common during acute attacks and like K+ is the result of shifts into the intracellular compartment.
Excess thyroid hormone may predispose to paralytic episodes by increasing Na+/K+ ATPase activity. The activity of this pump is likely to be increased further by catechols which are typically increased in this setting. The underlying cause of thyrotoxicosis is most commonly Graves’ disease but can also be a solitary thyroid adenoma, a thyroid-stimulating hormone-secreting pituitary adenoma, or abuse of thyroid hormone. Iodine-induced thyrotoxicosis (Jod-Basedow syndrome) and associated hypokalemic periodic paralysis has been reported following the administration of iodine-containing radiocontrast agents, amiodarone, and kelp supplements. “Dream Shape” and “Ever Youth” are two herbal medications used for weight reduction reported to cause iodine-induced thyrotoxicosis without periodic paralysis.
The acute attacks are treated with intravenous KCl and propranolol. It is important to administer KCl in non-dextrose-containing solutions since glucose will stimulate insulin release potentially exacerbating the movement of K+ into cells. In order to minimize the likelihood of rebound hyperkalemia, K+ should be given very cautiously and slowly at doses <10 mmol/hour. The goal of potassium administration is not to correct the hypokalemia to the normal range but to increase to a plasma [K+] of no more than 3.4 mEq/L, and then to discontinue potassium administration in order to avoid severe hyperkalemia, while continuing propranolol as the therapy of choice in this setting. Propranolol (a nonspecific β-adrenergic blocker) blocks the effects of catecholamines and inhibits the peripheral conversion of T4 to T3. The definitive treatment is to remove the underlying cause of thyrotoxicosis. Periodic paralysis does not recur once the patient is euthyroid.
The familial form of hypokalemic periodic paralysis is inherited as an autosomal dominant disorder and has similar clinical features as the acquired form. Notable differences include a younger age at presentation (usually <20 years), an equal male-female distribution, and is mostly seen in Caucasians. The familial disorder is most commonly due to mutations in the muscle calcium channel α-1 subunit gene (CACNA1S) on chromosome 1q3132. The α-1 subunit of the calcium channel serves as the pore for movement of calcium into the T tubule and also contains the dihydropyridine binding site. Mutations of this subunit reduce the calcium current into the T tubule. The precise mechanism by which impaired function of the calcium channel dihydropyridine receptor causes the influx of K+ into muscle cells is not entirely clear. A smaller number of cases have been localized to mutations in the skeletal muscle sodium channel SCN4A and the R83H mutation in the K+ channel subunit gene KCNE3. Mutations in these genes are not found in patients with thyrotoxic hypokalemic periodic paralysis (38).
The clinical phenotype of patients, together with the pattern of response to therapy, has been shown to differ depending on the location of the mutation. The carbonic anhydrase inhibitor, acetazolamide, is typically an effective therapy in reducing the number of attacks in patients with the familial disorder. The effectiveness of the drug has been attributed to induction of metabolic acidosis, which would in turn limit the intracellular shift of K+ into cells. However, recent work in an animal model of periodic paralysis suggests the beneficial effects of the drug is actually due to a direct stimulatory effect on Ca2+-activated K+ channels and not the induction of metabolic acidosis (39). While generally effective, a small number of patients given acetazolamide may demonstrate an exacerbation of symptoms (40). In this regard, the R83H mutation in the K+ channel subunit gene KCNE3 reduces single-channel conductance as compared to wild-type channels (41). This decrease in conductance is further reduced under conditions of low pH. It has been suggested that this mutation may explain the tendency for paralytic attacks to occur postexercise since skeletal muscle intracellular pH drops during this period. In addition, this sensitivity to acidosis could provide an explanation for why some patients with this disease worsen after acetazolamide therapy.
Another condition that needs to be considered when evaluating a hypokalemic patient with paralysis is classical distal renal tubular acidosis (dRTA) (42). Muscle paralysis in this disorder can begin insidiously with weakness evolving gradually over a 24- to 48-hour time period to complete flaccid quadriplegia. Attacks of flaccid paralysis in dRTA have been referred to as “RTA crisis” by some authors because this striking clinical manifestation may result in the clinician overlooking the underlying cause. Most of these cases have occurred in patients with dRTA that is idiopathic in origin or as a manifestation of Sjögren’s syndrome in which autoantibody generation prevents the trafficking of the H+-ATPase to the apical membrane.
Extrarenal K+ Loss from the Body
Cutaneous loss of K+ sufficient to cause hypokalemia is uncommon but may occur in the setting of intense exercise in a hot humid environment. Under these conditions, large volumes of sweat can be lost each day.
Sweat rates of approximately 2 L/hour were found in a study of football players who were members of a National Collegiate Athletic Association Division II team undergoing preseason training (43). The players practiced 4.5 hours/day and therefore were losing 9 L of sweat per day. This rate of sweat loss was no different whether practices were conducted in half pads or full pads. Body weight only declined between 1 and 2 kg depending on the time of day body weight was measured since the players were allowed free access to water.
Sweat rates of approximately 2 L/hour were found in a study of football players who were members of a National Collegiate Athletic Association Division II team undergoing preseason training (43). The players practiced 4.5 hours/day and therefore were losing 9 L of sweat per day. This rate of sweat loss was no different whether practices were conducted in half pads or full pads. Body weight only declined between 1 and 2 kg depending on the time of day body weight was measured since the players were allowed free access to water.
A similar sweat volume loss (2.1 L) was found in elite soccer players studied during a single 90-minute preseason training session (44, 45, 46). As observed in the football players, body weight declined by a lesser amount (1.2 kg) secondary to fluid intake. Na+ and K+ concentration was measured in sweat samples obtained from absorbent sweat pads placed on the chest, forearm, back, and thigh in the soccer players. Sweat Na+ concentration was 30 mmol/L and total sweat Na+ loss was 67 mmol. The respective values for K+ were 3.58 mmol/L and 8 mmol. There are two points worth emphasizing in these studies. First, sweat losses can be substantial in well-trained athletes during exercise. Despite the free availability of water, these athletes remain mildly dehydrated in the immediate postexercise period. Secondly, while the K+ concentration is low in sweat, significant total body K+ loss can develop when sweat volume is high.
There is an impression by many that cramps that develop in athletes are related to loss of K+ in sweat. To address this issue sweat Na+ and K+ losses were measured in Division I collegiate football players undergoing twice-daily practice sessions (47). Values obtained were compared between players with a history of heat cramps (C) and teammates with no history of cramping (NC). In a 2.5-hour practice session, sweat loss was similar between the two groups (4.0 l [C] vs. 3.5 l [NC]). Sweat K+ was also similar in the two groups but sweat Na+ was two times higher in players with a history of heat cramps (54 vs. 25 mmol/L). These data suggest it is large acute Na+ and fluid loss rather than K+ loss that is associated with a tendency to develop cramps during exercise.
Gastrointestinal loss is a common cause of hypokalemia and is generally due to diarrhea. Secretory diarrhea is generally believed to be caused by one of two processes which can either occur alone or both together (48, 49). First, there can be inhibition of active intestinal NaCl and NaHCO3 reabsorption and second, there can be stimulation of active chloride secretion which is then followed by passive secretion of an equal amount of Na+ so as to maintain electrochemical balance. In both of these instances, the stool electrolyte content is similar to plasma with high concentration of NaCl and much lower K+ concentration. The sodium salts in stool cause an isotonic increase in stool water output such that the fecal content of sodium salts roughly parallels the volume of diarrhea. Despite the low K+ concentration in fecal fluid, significant total body K+ losses can occur in the setting of large stool volumes.
Severe K+ depletion due to secretory diarrhea has been the subject of recent case reports. One patient with neurofibromatosis type 1 presented with the syndrome of watery diarrhea, hypokalemia, and achlohydria due to oversecretion of vasoactive intestinal polypeptide (VIP) (50). A neuroendocrine tumor composed of pheochromocytoma and ganglioneuroma was responsible for the VIP production. A second patient presented with hypokalemia complicated by rhabdomyolysis due to oversecretion of pancreatic polypeptide (PP) from a pancreatic islet tumor (51). PP normally exerts an inhibitory effect on gastric emptying and upper gastrointestinal motility but has been reported to cause a watery diarrhea syndrome similar to that as a VIPoma. In this patient severe hypokalemia developed with minimal gastrointestinal complaints. The authors speculated that the inhibitory effect of PP on gut motility may have attenuated the development of watery diarrhea. Hypokalemia and volume depletion can also occur in association with villous adenomas, a symptom complex referred to as the McKittrick-Wheelock syndrome (52).
Abnormalities in K+ transport have not previously been known to be the primary cause of secretory diarrhea. The first such case has recently been described in an elderly woman with colonic pseudo-obstruction (Ogilvie’s syndrome) (53). One week after undergoing surgical treatment of a hip fracture, a 78-year-old woman developed diarrhea, hypokalemia, and a markedly dilated colon. The workup excluded identifiable causes of intestinal obstruction and diarrhea. Fecal fluid was collected on multiple occasions and electrolyte content was measured. In contrast to the high Na+ concentration (101-137 mEq/L) and low K+ concentration (16-51 mEq/L) typically found in various causes of secretory diarrhea, fecal electrolyte concentration in this patient was reversed. Fecal K+ concentration ranged from 130 to 170 mEq/L while values for Na+ concentration varied between 4 and 15 mEq/L. In addition, increased stool weight was accompanied by a proportionate increase in fecal K+ output while stool Na+ changed very little. Measurement of the rectosigmoid potential difference in this patient was -13 mV (lumen negative). When the data were interpreted in the context of the Nernst equation, the authors concluded there was evidence of active K+ secretion along with active Na+ reabsorption across the colonic mucosa. The patient’s pseudo-obstruction spontaneously resolved over a 14-week period.
As in the kidney, K+ is actively absorbed and secreted in the colon whereas in the small intestine K+ movement is strictly diffusional. Colonic K+ absorption occurs via the colonic isoform of H+/K+ ATPase, located
on the luminal membrane, while K+ secretion occurs via a luminal channel (54, 55). Dietary K+ restriction increases colonic K+ absorption while secretion predominates with high dietary K+ intake. In patients with end-stage renal disease, increased secretion of K+ by the colon is an important adaptation to K+ homeostasis. Recent studies indicate colonic K+ secretion is mediated by the BK channel (56). This channel is a high conductance channel that is activated by calcium and is located exclusively in the colonic crypts and not on surface cells. Increased expression of this channel may mediate the enhanced colonic K+ secretion in patients with end-stage renal disease.
on the luminal membrane, while K+ secretion occurs via a luminal channel (54, 55). Dietary K+ restriction increases colonic K+ absorption while secretion predominates with high dietary K+ intake. In patients with end-stage renal disease, increased secretion of K+ by the colon is an important adaptation to K+ homeostasis. Recent studies indicate colonic K+ secretion is mediated by the BK channel (56). This channel is a high conductance channel that is activated by calcium and is located exclusively in the colonic crypts and not on surface cells. Increased expression of this channel may mediate the enhanced colonic K+ secretion in patients with end-stage renal disease.
Bowel cleansing solutions can occasionally be associated with perturbations in serum electrolyte levels to include hypokalemia, hyperphosphatemia, hypocalcemia, and hypernatremia. Risk factors for these disturbances include advanced age, the presence of bowel obstruction, poor gut motility, and unrecognized renal disease. Use of oral sodium phosphate for bowel preparation has recently been linked to the development of acute renal failure characterized histologically by widespread deposition of calcium phosphate crystals (57). A recent study randomly allocated 100 consecutive patients who were to undergo colonoscopy to either receive sodium phosphate or a glycol-electrolyte solution (Golytely, Braintree Laboratories) for bowel cleansing (58). Eleven patients received sodium phosphate despite the presence of factors that would have identified them as being at risk for complications. In six of these high-risk patients, the serum phosphate doubled and hypokalemia developed in four. In subjects without risk factors, mild hyperphosphatemia developed in 39% and hypocalcemia developed in 5%. In the glycol-electrolyte solution-treated patients, only mild and clinically insignificant changes in serum electrolyte values occurred. The glycol-electrolyte solution is a nonabsorbable and osmotically balanced solution and has virtually no net effect on electrolyte absorption or secretion.
Severe hypokalemia can result from K+ binding in the gastrointestinal tract. A serum K+ concentration of 0.9 mmol/L was found in a 3-year-old girl following several days of oral and rectal administration of bentonite given as a home remedy for the treatment of constipation (59). Bentonite, also called montmorillonite or fuller’s earth, is a type of clay primarily composed of hydrated aluminum silicate. Clay eating (geophagia) can be a manifestation of pica and has been reported to cause hypokalemic paralysis during pregnancy and in the postpartum period (60, 61).
Renal Potassium Wasting
The elaboration of aldosterone and distal delivery of Na+ and water are two important factors in the renal excretion of K+. Although increased distal delivery of Na+ and water and increased aldosterone activity can each stimulate renal K+ secretion, under normal physiologic conditions, these two determinants are inversely related. It is only under pathophysiologic conditions that distal Na+ delivery and aldosterone become coupled. In this setting, renal K+ wasting will occur (Fig. 5-2). When treating patients who are hypokalemic as a result of renal K+ wasting, it must be determined whether there is a primary increase in mineralocorticoid activity or a primary increase in distal Na+ delivery (62).
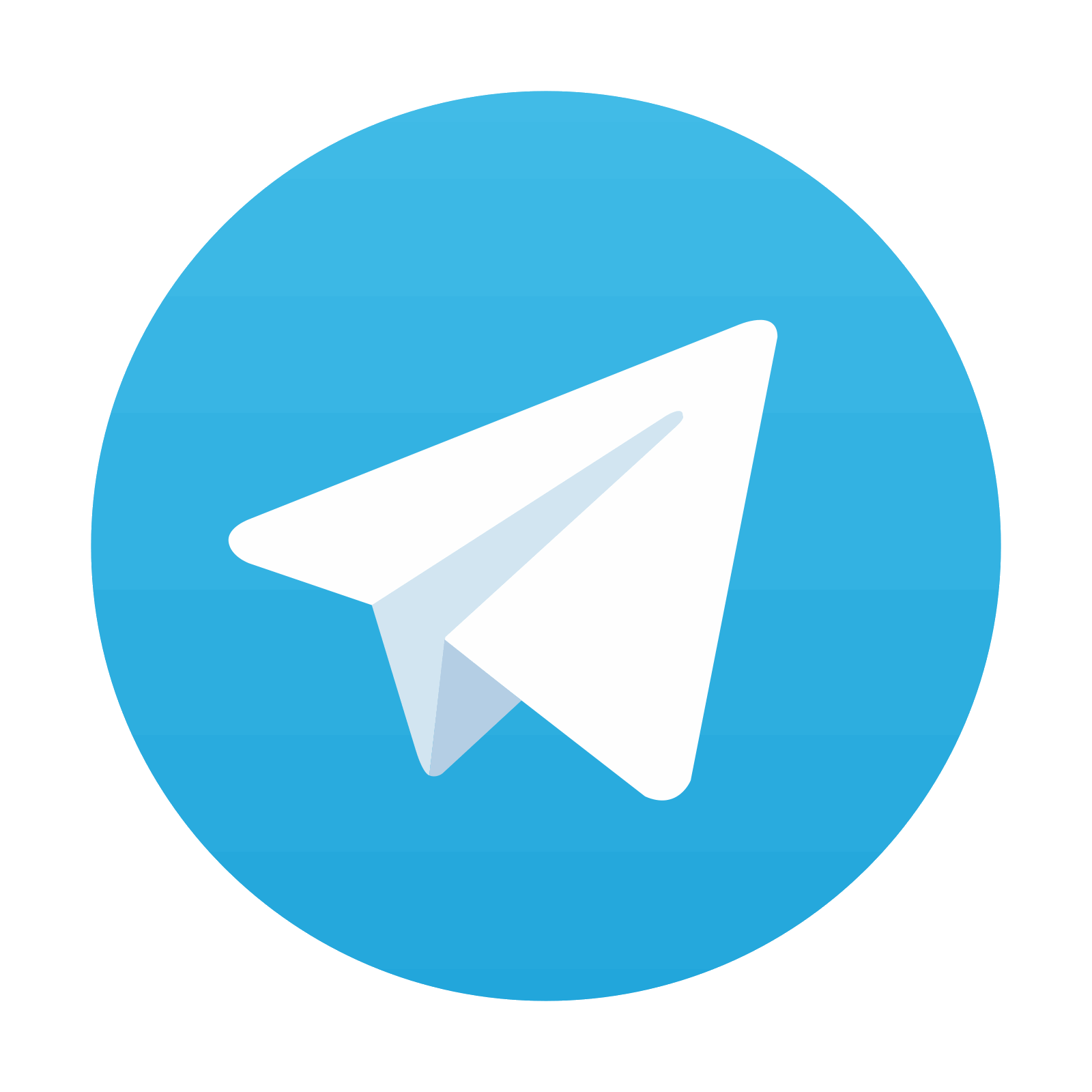
Stay updated, free articles. Join our Telegram channel
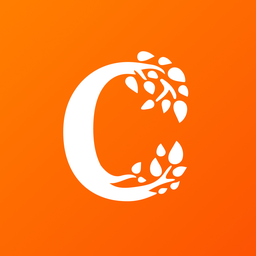
Full access? Get Clinical Tree
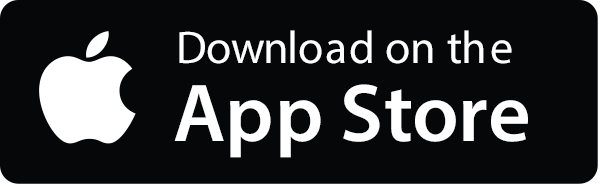
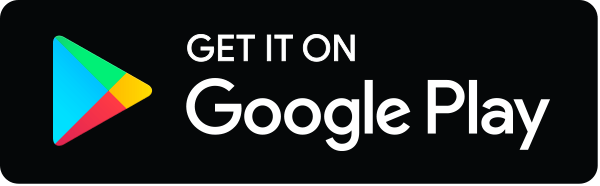