Disorders of Calcium, Phosphorus, Vitamin D, and Parathyroid Hormone Activity
Mordecai M. Popovtzer
Serum Calcium Concentration
The calcium ion is essential to any physiologic phenomena, including preservation of the integrity of cellular membranes, neuromuscular activity, regulation of endocrine and exocrine secretory activities, blood coagulation, activation of the complement system, and bone metabolism.
Total Serum Calcium Concentration
The normal range for total serum calcium must be established for each laboratory and varies according to the method used. Total serum calcium is divisible into protein-bound and ultrafiltrable (diffusible) calcium (Fig. 6-1).
PROTEIN-BOUND CALCIUM
Approximately 40% of total calcium is bound to serum proteins, and 80% to 90% of this calcium is bound to albumin. Variations in serum protein alter proportionately the concentration of the protein-bound and total serum calcium. An increase in serum albumin concentration of 1 g/dL increases protein-bound calcium by 0.8 mg/dL, whereas an increase of 1 g/dL of globulin increases protein-bound calcium by 0.16 mg/dL. Thus, it is obvious that changes in total serum calcium concentration cannot be used for the assessment of the effect on bound calcium concentration unless the changes in albumin and globulin concentrations also are determined. Marked changes in serum sodium concentration also affect the protein binding of calcium.
Hyponatremia increases, whereas hypernatremia decreases protein-bound calcium. Changes in pH also affect protein-bound calcium, and an increase or decrease of 0.1 pH increases or decreases protein-bound calcium by 0.12 mg/dL. Respectively, in vitro, freezing and thawing serum samples may decrease the binding of calcium as well.
ULTRAFILTRABLE (DIFFUSIBLE) CALCIUM
Serum ultrafiltrable calcium is obtained by applying pressure on serum against a semipermeable membrane. Thus, serum water is forced across the membrane, and the ultrafiltrate is analyzed for calcium concentration and then corrected for total serum solids. The samples must be handled anaerobically, because changes in pH may affect calcium binding. Under normal conditions, ultrafiltrable calcium constitutes 55% to 60% of the total serum calcium.
FREE (IONIZED) CALCIUM
The biologically active component of diffusible calcium is ionized calcium. Flow-through and static ion exchange electrodes, which function similarly to conventional pH electrodes, are used. Serum-ionized calcium concentration in normal subjects ranges from 4.0 to 4.9 mg/dL, or 47% of total serum calcium. The samples have to be handled anaerobically because changes in pH alter the concentration of ionized calcium. Determinations are best made on freshly separated serum, because heparin creates complexes with calcium, and the presence of fibrin may interfere with the structural integrity of the porous membrane used in the procedure. Storage of serum in oil does not prevent changes in pH, because carbon dioxide dissolves readily in oil. An increase in serum pH of 0.1 unit may cause a decrease in ionized calcium of 0.16 mg/dL (1, 2). As with ultrafiltrable calcium, freezing and thawing of serum may alter the level of ionized calcium.
COMPLEXED CALCIUM
The nonionized portion of diffusible or ultrafiltrable calcium is called complex calcium. The calcium complexes are formed with bicarbonate, phosphate, and acetate. The amount of complexed calcium is measured indirectly by subtracting the ionized calcium (47%) from the ultrafiltrable calcium (60%) and thus equals about 13% of total serum calcium. The complexed calcium has been found to be increased twofold in patients with uremia.
CYTOSOLIC CALCIUM
Cytosolic calcium can be measured by loading the tested cells with a fluorescent probe such as indo-1-acetoxymethyl ester and exciting the cells at 350 nM. The ratio of fluorescence emission at 410 nM to that at 490 nM is used as an index of free intracellular calcium. The normal concentration of cytosolic calcium is 100 nM/L, which is 10,000-fold lower than the concentration of extracellular calcium. The very steep gradient is maintained by an energy-driven calcium pump, known as the plasma membrane Ca2+ ATPase (PMCA). In certain types of cells, a Na+/Ca2+ exchanger, energized by a Na+-gradient, helps drive cytosolic calcium into the extracellular space. Part of cellular calcium is sequestered in intracellular organelles, including endoplasmic reticulum, sarcoplasmic reticulum (SR) in muscle cells and in mitochondria. The calcium-dependent intracellular signaling generally requires a 10-fold increase in cytosolic calcium. With each heartbeat, the cytosolic calcium concentration in cardiac myocytes is elevated 10-fold, from a resting level of 100 to 1,000 nM. Likewise, in other signaling events such as T-cell activation, which triggers the transcription of interleukin-2, a 10-fold increase in cytosolic calcium serves as the signal for the response. Elevation of cytosolic calcium is mediated by activation of calcium channels, which allows passive calcium flux down its electrochemical gradient.
Calcium plays an important role in cardiac coupling of excitation and contraction. The lasting phase of cardiac action potential is maintained by L-type calcium channels, whereby extracellular calcium influx into the intracellular space activates calcium-sensitive channels of SR. Relatively small amounts of calcium entering the cell via the L-type channel activate a small number of SR calcium channels. This leads to calcium release from SR and a marked increase in intracellular calcium concentration, termed “calcium-induced calcium release.” Calcium removal from the cytoplasm occurs via active transport back to SR by calcium pump, Ca2+ ATPase. A smaller amount, the same amount that entered the cell through L-type calcium channel, is extruded into the extracellular space via the sodium-calcium exchanger (NCX). Glycosides, the drugs known for positive inotropic effect, act by inhibiting the sodium pump (Na+/K+ ATPase), increase intracellular sodium load in the myocytes. Subsequent activation of NCX leads to sodium extrusion and calcium into the myocyte cytosol. Glycosides represented the only effective therapy for congestive heart failure for a long time (3).
Serum Phosphorus Concentration
Serum phosphorus occurs in two forms, organic and inorganic. Organic phosphorus is composed entirely of phospholipids bound to proteins. The inorganic fraction is the principal circulating form of phosphorus and is routinely assayed for clinical uses. About 90% of inorganic phosphorus is ultrafiltrable. About 53% of the ultrafiltrable inorganic phosphorus in serum is dissociated with a 1:4 ratio of H2PO4– to HPO42-; the remainder of ultrafiltrable phosphate is in the form of salts of sodium, calcium, and magnesium. During marked hyperphosphatemia (8-10 mg/dL of serum), a significant portion of phosphate forms colloidal complexes with calcium that are rapidly removed from the circulation.
Studies propose that an increase in serum phosphorus leads to a reciprocal fall in serum calcium, so that the product of both ions remains constant. The assumption was the solubility equilibrium exists between the bone and the extracellular fluid (ECF). However, this appears to be an oversimplification of a more complex equilibrium. An inverse relationship between serum calcium and phosphorus is present only under extreme changes in serum phosphorus; for example, a decrease in serum calcium occurs following an acute rise in serum phosphorus. By contract, this relationship does not hold for acute changes in serum calcium, because a rapid increase in calcium leads to a rise rather than a fall in serum phosphorus before any changes in urinary phosphorus occur (2). This effect may be caused by the release of phosphorus from cells. Serum phosphorus displays circadian variations. Serum phosphorus levels reach a nadir early in the morning, with an increase to plateau at 4 p.m., followed by a further increase to a peak at 1 to 3 a.m.
Serum phosphorus concentration is also influenced by age. In adults, the normal concentration ranges from 2.5 to 4.0 mg/dL, whereas in children it ranges from 4 to 6 mg/dL. The level of alkaline phosphatase in children is higher than in adults. These age differences are probably related to different rates of skeletal growth. Serum phosphorus decreases during hyperventilation and alkalosis and increases during acidosis. Serum phosphorus also varies directly with its content in the diet. Administration of glucose causes a fall in serum phosphate because of the flux of phosphate into cells with the phosphorylation of glucose. The administration of insulin and epinephrine also reduces serum phosphorus concentration. Hypophosphatemia occurring in sepsis and acute myocardial infarction may result from the release of epinephrine into the circulation.
A recent large population study showed that serum phosphorus is only weakly related to dietary phosphorus intake and/or phosphorus-rich foods, suggesting that other factors determining serum phosphorus concentration remain to be defined (4). This finding may bear on another earlier population study that showed that higher serum phosphorus levels, even within the normal range, had been associated with increased risk of cardiovascular events in patients with normal kidney function (5). The clinical relevance of these and other population-wide studies is uncertain.
Calcium and Phosphorus Balance
Total body calcium ranges from 1.0 to 1.5 kg and that of phosphorus from 0.5 to 0.8 kg. Ninety-nine percent of total calcium and 85% of total phosphorus are stored in the skeleton. Only 1% of both are in the ECF, and the remainder is intracellular.
DIETARY CALCIUM AND PHOSPHORUS
Dietary calcium and phosphorus intake varies considerably. In general, balanced diets provide from 800 to 1,200 mg of calcium and from 800 to 1,500 mg of phosphorus per day. The minimum daily requirement of calcium is 400 to 500 mg, and an intake below this amount may cause a negative calcium balance. Dietary calcium can be reduced to about 200 mg by the exclusion of dairy products (6), and boiling of vegetable causes a loss of 25% of their calcium content. It has become common to enrich bread with powdered milk to increase the amount of calcium in the diet. Drinking water is also a source of calcium; “soft” water has 1 to 3 mg/dL of calcium, and “hard” water has 3 to 10 mg/dL. Human diets, almost without exception, contain more phosphorus than calcium, because phosphorus is present in almost all foodstuffs. The amount of calcium and phosphorus in various foods is shown in Table 6-1.
INTESTINAL ABSORPTION OF CALCIUM
Calcium is absorbed along the small intestine, more in the duodenum and proximal jejunum than in the ileum (6, 7, 8). The absorption of calcium is completed within 4 hours after its oral intake (8, 9). Calcium absorption in the gastrointestinal (GI) tract occurs via two transport processes (10, 11, 12, 13). Transcellular calcium absorption, which is saturable and physiologically regulated, follows three steps: (a) luminal entry into mucosal cells through apical calcium channels; (b) binding to a protein carrier, calbindin 9k, and transfer to the serosal side; and (c) extrusion from the cell by an active process at the basolateral side by Ca2+ ATPase (calcium pump) and also most likely, by NCX. Increasing body demands for calcium activate maximally the transcellular transportation. Paracellular calcium absorption is nonsaturable and is driven by concentration gradients between luminal and serosal spaces. Thus, the rate of absorption depends primarily on calcium concentration in the lumen. This pathway of absorption predominates in the distal small bowel. The paracellular absorption route traverses the apical tight junctions of the mucosal cells; therefore, changes in permeability in these sites also may affect the rate of transport. In contrast to the paracellular pathway, the transcellular route represents an actively controlled mechanism of calcium reabsorption. Identification of epithelial calcium channels TRPV6 and, to a lesser extent, TRPV5 advanced our understanding of transcellular calcium reabsorption (14). It appears that TRPV5/6 is regulated by vitamin D. ECaC was first cloned from a rabbit kidney cortex. In addition to the kidney, TRPV messenger ribonucleic acid (mRNA) is expressed in the small intestine as well.
Immunohistochemical staining located the channel protein in the apical membrane of renal epithelial cells and the brush-border membrane of duodenal and jejunal villi. TRPV mRNA and protein abundance are decreased in states of vitamin D deficiency and increased with vitamin D repletion. TRPV is the rate-limiting factor in active calcium absorption by regulating the apical entry of calcium into the epithelial cells. The TRPV family consists of two homologous species; TRPV refers mainly to the renal channel, whereas ECaC2 is mainly the intestinal channel. Genomic cloning showed that TRPV5 and TRPV6 are products of distinct genes; both are juxtaposed on chromosome 7q35, suggesting evolutionary gene duplication. TRPV6 has been cloned from rat, mouse, and human intestines.
The absorption of calcium becomes more efficient with low calcium intake, thus ensuring that adequate amounts of calcium are delivered to the body. This process of adjustment to low calcium intake, which is not entirely understood, has been termed “adaptation.” Younger persons exhibit this phenomenon of adaptation better than older individuals. The absorption of calcium also increases in direct proportion to the requirements; for example, calcium absorption increases during pregnancy and depletion of total body calcium.
Table 6-1 Calcium and Phosphorus Content in Different Foods | ||||||||||||||||||
---|---|---|---|---|---|---|---|---|---|---|---|---|---|---|---|---|---|---|
|
Oral calcium may be complexed, chelated, or precipitated in the GI tract by a variety of substances that render it unavailable for absorption. These substances include phytate, oxalate, and citrate. Certain drugs, including colchicine, fluoride, theophylline, and glucocorticoids, also interfere with calcium absorption. Rapid motility or shortening of the length of the GI tract may diminish the absorption of calcium as well. Decreased calcium absorption has been observed with protein depletion both in human subjects and in rats. A deficiency of the specific calcium-binding protein in the intestinal mucosal cells has been proposed as the mechanism accounting for this failure of calcium transport.
In the absence of oral intake, calcium continues to be excreted in the feces and a negative calcium balance ensues. Thus, it is apparent that some of the fecal calcium is derived from intestinal secretion. Using an intravenous tracer method, the daily calcium secretion has been estimated to be on the order of 150 mg/day. This amount does not change during an intravenous load of calcium.
Net calcium absorption (dietary calcium minus fecal calcium) can be determined by maintaining the patient on a constant diet and collecting stools. This balance method is time consuming, because it requires an equilibration period of several days followed by a collection period of several days. The results may be expressed in absolute values, where the net calcium absorption is the difference between calcium intake and calcium fecal excretion. Alternatively, the results can be expressed as fractional calcium absorption, as shown in the following formula:

INTESTINAL ABSORPTION OF PHOSPHORUS
About 50% to 65% of dietary phosphorus is absorbed, mostly in the jejunum. Evidence from in vitro studies indicated that phosphorus absorption is an active process. The active process is sodium coupled and saturable.
Phosphate is transported across the mucosal brush-border membrane against an electrochemical gradient. This active transport is sodium dependent and is driven by a sodium gradient generated and maintained by the activity of Na+/K+ ATPase at the basolateral membrane. A sodium-phosphate (NaPi) cotransporter has been cloned from the mouse small intestine and designated as a type IIb (NaPiIIb) cotransporter in analogy to a type IIa (NaPiIIa) cotransporter cloned from the kidney (15). Type IIb is located in human chromosome 4, whereas type IIa (NaPiIIa) is located in human chromosome 5. NaPiIIb protein was localized in the intestinal brush-border membrane vesicles by Western analysis. The type IIb (NaPiIIb) cotransporter was characterized by functional studies in complementary RNA (cRNA)-injected oocysts and shown to have the features of intestinal NaPi transport. Type IIb-mediated NaPi cotransport is enhanced by more acidic pH as opposed to the renal type IIa transporter, which is stimulated by more alkaline pH. It has been shown that 1,25(OH)2D increases phosphorus transport by stimulating NaPiIIb cotransporter. Experimental studies in rodents demonstrated age-dependent response to 1,25(OH)2D. In suckling animals, vitamin D increased NaPiIIb gene expression and protein abundance, whereas in adult rodents 1,25(OH)2D increased protein abundance without changes in gene expression of the cotransporters in the small intestine (16). Similarly, low-phosphorus diet-stimulated intestinal phosphorus absorption is associated with NaPiIIb protein abundance without changes in gene expression. This response is independent of vitamin D (17). Nicotinamide-induced inhibition of intestinal phosphorus absorption is associated with a decrease in NaPiIIb protein abundance in brush-border membrane vesicles.
There is, however, a linear correlation between phosphorus intake and net absorption. This reflects the passive paracellular pathway of transport, which is determined by concentration gradients of phosphorus across the intestinal mucosa. In contrast to findings in animals, high phosphate intake in humans does not seem to cause a decrease in calcium absorption. Rather, the presence of phosphate in the diet is necessary for calcium absorption. Phosphate absorption may be decreased by a high calcium intake or ingestion of aluminum hydroxide antacids, which bind phosphorus in the bowel, thereby inhibiting its absorption. Similarly, sevelamer (Renagel), lanthanum, and sucroferric oxyhydroxide can reduce intestinal phosphate absorption.
URINARY EXCRETION OF CALCIUM
The urinary excretion of calcium varies considerably in normal subjects, but the oral intake only modestly affects the daily urinary excretion of calcium. The upper normal range of calcium excretion per day has been estimated to be <300 mg for men and <250 mg for women, or 4 mg/kg body weight. Unlike the response to
a low-sodium diet, institution of a diet very low in calcium does not lead immediately to a substantial reduction in urinary calcium. However, in clinical states of protracted calcium depletion, such as in patients with intestinal malabsorption and osteomalacia, urinary excretion of calcium may be reduced to 50 mg/day or less.
a low-sodium diet, institution of a diet very low in calcium does not lead immediately to a substantial reduction in urinary calcium. However, in clinical states of protracted calcium depletion, such as in patients with intestinal malabsorption and osteomalacia, urinary excretion of calcium may be reduced to 50 mg/day or less.
Only ultrafiltrable calcium crosses the glomerular capillary walls and is then partially reabsorbed by the tubular epithelial cells. In adults, 97% to 99% of filtered calcium is reabsorbed. The tubules reabsorb ionized calcium more easily than complexed calcium, which accounts for the fact that the proportion of ionized calcium in the urine is only 20% of the total, the remainder being complexed calcium. The calcium complexes contain many anions such as citrate, sulfate, phosphate, and gluconate. Citrates in the urine bind calcium most powerfully. Sixty percent of calcium is chelated with citrate at a neutral pH in 1 L of urine containing 100 mg of calcium and 480 mg of citrate; this fraction falls to 40% at a pH of 5.0.
Urinary excretion of calcium is influenced by oral intake and urinary excretion of sodium. Thus, any attempt to assess urinary calcium excretion must take into account the oral intake and excretion of sodium.
It has also been found that factors that affect the renal excretion of sodium, such as ECF volume expansion, similarly alter the renal excretion of calcium. Chronic expansion of ECF volume with mineralocorticoid hormone increases the urinary excretion of calcium as well.
It has been estimated that 50% to 70% of filtered calcium is reabsorbed in the proximal nephron, 30% to 40% is reabsorbed between the end of the accessible part of the proximal tubule and the distal tubule (DT), and the remaining 10% is reabsorbed in the distal nephron (13, 18). Micropuncture studies have demonstrated that sodium and calcium exhibit very similar absorptive characteristics in the proximal tubule. In the thick ascending limb (TAL) of the loop Henle, the absorption of both ions follows the same direction. Lumen-positive voltage is the driving force for calcium reabsorption in this segment. Furosemide abolishes the transepithelial potential; therefore, it reduces calcium absorption in parallel with reduced sodium reabsorption. Parathyroid hormone (PTH) reduces urinary excretion of calcium but augments urinary excretion of sodium.
Recent studies have provided more detailed insight into the tubular mechanisms underlying calcium transport along various nephron segments. The main fraction of filtered calcium is reabsorbed via paracellular passive flux that is driven by an electrochemical gradient in the proximal tubule and in the TAL, accounting for 80% to 90% of total filtered calcium. Epithelia permit selective and regulated flux from apical to basolateral surfaces by paracellular flux between cells or transcellular passage through cells. Tight junctions constitute the route for paracellular conductance in TAL for divalent cations, whereas the epithelial calcium channel TRPV5, in the distal convoluted tubule (DCT) and connecting tubule (CNT) constitute the apical entry mechanism of active transcellular calcium transport. Two conditions have to be met in TAL for paracellular reabsorption of divalent cations—the transepithelial voltage must be oriented lumen positive, and the paracellular route must allow divalent cation conductance. In this regard, alterations in transepithelial NaCl reabsorption are a determinant of divalent cation reabsorption by means of changes in transepithelial voltage generation. The observations that humoral factors (e.g., PTH) and basolateral concentration changes of divalent ions without changes in transepithelial voltage alter calcium reabsorption suggest a selective effect on paracellular permeability with specificity to divalent cations.
Claudins are members of tight-junction membrane proteins that act both as paracellular pores and as barriers that express selectivity to ions. Claudin-16 (originally paracellin 1) and claudin 19 are expressed in the thick ascending loop of Henle (TALH). Both facilitate paracellular absorption of divalent ions. In vitro and in vivo studies have shown that both claudin-16 and claudin-19 play a role in paracellular reabsorption of calcium and magnesium. The lumen-positive potential is proposed to be the driving force for divalent ion, Mg2+ and Ca2+, reabsorption. In this regard, mutations of claudin-16 and claudin-19 genes cause autosomal recessive familial hypomagnesemia, hypercalciuria, and nephrocalcinosis (FHHNC).
Recently, a new claudin, claudin-14, was identified in TALH; it is associated with kidney stone disease. The underlying mechanism for hypercalciuria and stone formation is inhibition of claudin-16 by claudin-14. Sequence variants in claudin-14 gene are associated with kidney stones and low bone density.
Claudin-14 is upregulated by activation of calcium sensing receptor (CaSR). Upregulation of CaSR as in the case of hypercalcemia leads to activation of claudin-14 and inhibition of claudin-16, leading to hypercalciuria resembling the phenotype of FHHNC (19, 20, 21, 22).
Extracellular CaSR plays a key role in calcium and magnesium reabsorption in TAL. Extracellular calcium and other cations (e.g., Mg2+) activate mechanism(s) that control their paracellular tubular transport by acting on the basolateral CaSR that recognizes those cations as their extracellular ligands. CaSR is a G protein-coupled receptor that leads to a transient rise in intracellular calcium by activation of phospholipase C, hydrolysis of phosphatidylinositol 4,5- to biphosphate, and increased by the formation of inositol triphosphate and diacylglycerol. CaSR is expressed by the cells of the TAL and plays a role in the normal regulation of calcium
absorption in the nephron segment. Thus, normal or high calcium concentration is likely to be “sensed” by CaSR activating a signal transduction cascade and leading to reduced paracellular calcium reabsorption. Conversely, low calcium concentration (i.e., hypocalcemia) would fail to activate the signal transduction pathway, thus leading to abnormally avid calcium reabsorption and hypocalciuria. The latter also explains the hypocalciuria observed in patients with familial hypocalciuric hypercalcemia (FHH), in whom the gene that encodes the CaSR has undergone an inactivating mutation, leading to a defective receptor (23). Theoretically, the CaSR-initiated signaling could regulate calcium absorption by three putative mechanisms: (1) altering the permeability to calcium of the paracellular tight junction pathway, (2) changing the apical electrolyte transport that generated the TAL luminal electropositivity that is the driving force for calcium absorption, or (3) both.
absorption in the nephron segment. Thus, normal or high calcium concentration is likely to be “sensed” by CaSR activating a signal transduction cascade and leading to reduced paracellular calcium reabsorption. Conversely, low calcium concentration (i.e., hypocalcemia) would fail to activate the signal transduction pathway, thus leading to abnormally avid calcium reabsorption and hypocalciuria. The latter also explains the hypocalciuria observed in patients with familial hypocalciuric hypercalcemia (FHH), in whom the gene that encodes the CaSR has undergone an inactivating mutation, leading to a defective receptor (23). Theoretically, the CaSR-initiated signaling could regulate calcium absorption by three putative mechanisms: (1) altering the permeability to calcium of the paracellular tight junction pathway, (2) changing the apical electrolyte transport that generated the TAL luminal electropositivity that is the driving force for calcium absorption, or (3) both.
Polymorphism with the R990G allele, which results in gain of function of CaSR, has been reported. This polymorphism has been associated with increased susceptibility to hypocalciuria and renal stone formation (24).
The fine-tuning of tubular calcium reabsorption, which is crucial for the maintenance of calcium balance, is regulated by active transcellular calcium transport in distal nephron segments. The calcium channel TRPV5 plays an important role in the process (14).
Immunohistochemical analysis demonstrated the presence of TRPV5 in the apical membrane of late DCT2 and CNT of the kidney cortex. TRPV5 is believed to constitute the rate-limiting mechanism, the first step in active calcium reabsorption. Following apical entry into the cytosol, calcium is bound to a carrier protein (calbindin D28K) that translocates the cation to the transporters residing in the basolateral cell surface, the NCX and ATP-dependent calcium pump, the PMCA, which extrude the calcium to the extracellular basolateral space. The latter can be inhibited by calcium via the CaSR that resides at the basolateral membrane of DCT.
PTH and 1,25-dihydroxycolecalciferol (1,25[OH]2 D3) are the major regulators of TRPV5 in the distal nephron. Parathyroidectomy in rats leads to a fall in TRPV5 expression, as well as diminished calbindin D28K and NCX. PTH supplementation restores the expression of TRPV5. 1,25(OH)2D3 increases the expression of TRPV5, calbindin D28K, PMCA1b, and NCX, thus harmonizing enhanced calcium absorption.
Recent observations suggest that Klotho, the antiaging hormone, upregulates distal calcium reabsorption by two putative mechanisms. The Klotho gene encodes a single-pass transmembrane protein with a sequence similar to the β-glucosidase enzyme. The extracellular domain of Klotho, pKlothorotein, is shed and present in the circulation and tubular lumen, potentially functioning as a human factor. First, in response to low extracellular calcium levels, Klotho binds to Na+/K+ ATPase and translocates it to the plasma membrane. The increased sodium gradient generated by Na+/K+ ATPase drives the transepithelial transport of calcium by activating the basolateral NCX. Second, Klotho in the urine increases TRPV5 abundance on the luminal surface by hydrolyzing the N-linked extracellular sugar residues of TRPV5. Both mechanisms augment calcium influx from the lumen. FGF23 is involved mainly in phosphate metabolism. Interestingly, recent experimental findings have demonstrated that FGF23 increases the expression of the calcium channel, TRPV5, on the apical membrane of the DT. This suggests that FGF23 may enhance calcium reabsorption in DT, similarly to vitamin D, PTH, and Klotho (25).
Inhibition of NaCl uptake in DCT by thiazides that bind to NaCl cotransporter leads to hypocalciuria. Studies suggest that suppression of NaCl transport into the DCT cells results in hyperpolarization of the cell that activates the TRPV5, thus promoting calcium entry into the cytosol. In parallel, the thiazide-induced fall in intracellular sodium concentration facilitates the action of NCX to enhance the exit of calcium from the cytosol to the extracellular basolateral compartment. Also, amiloride-, triamterene-, and spironolactone-induced inhibition of sodium reabsorption produces hypocalciuria in a similar fashion. However, more recent studies suggest that the effect of thiazides on tubular calcium reabsorption is primarily mediated by increasing proximal tubule reabsorption due to hypovolemia induced by thiazides (26). WNK4 that is a negative regulator of NaCl cotransporter in the DT enhances TRPV5-medated calcium transport. Inactivating mutation of WNK4 causes familial hyperkalemic hypertension (PHAII, Gordon syndrome) and leads to reduced calcium reabsorption and hypocalciuria (27).
High extracellular pH stimulates the activity of TRPV5, whereas low pH suppresses its activity. Consequently, pH-dependent inhibition of ECaC1 in acidosis may contribute to renal calcium wasting in this condition (21).
Changes in the filtered load of calcium also may affect the excretion of this ion. Thus, hypocalcemia is associated with a low urinary calcium excretion, regardless of its cause. A micropuncture study in dogs demonstrated that elevation of plasma calcium from a low to a normal level inhibits calcium reabsorption in the loop of Henle independent of PTH. The renal capacity to excrete calcium may be severely compromised by a reduction in glomerular filtration rate (GFR) and ECF volume depletion. The reduced absolute and fractional excretion of calcium in early chronic renal failure when the GFR is only moderately reduced is not well understood (28, 29). Two factors might contribute to this observation: secondary hyperparathyroidism
and abnormalities of vitamin D metabolism with reduced intestinal absorption of calcium (28). In more advanced renal failure, fractional excretion of calcium is enhanced and correlates with the fractional clearance of sodium, suggesting that the renal handling of both ions may be altered by a similar mechanism at this stage of renal insufficiency (28).
and abnormalities of vitamin D metabolism with reduced intestinal absorption of calcium (28). In more advanced renal failure, fractional excretion of calcium is enhanced and correlates with the fractional clearance of sodium, suggesting that the renal handling of both ions may be altered by a similar mechanism at this stage of renal insufficiency (28).
Acute and chronic loads of phosphorus may decrease urinary excretion of calcium. It has been proposed that the reduced urinary calcium excretion is because of an increased deposition of mineral, either in the bone or in other tissues. The hypocalciuria following oral phosphates has been used in the treatment of renal calculi.
Phosphate depletion leads to an increased urinary excretion of calcium, although the mechanism for this effect remains to be defined. The possible role of secondary hypoparathyroidism was not supported by studies in animals in which parathyroidectomy did not alter substantially the hypercalciuric response to phosphate depletion. In rats, the hypercalciuric response to phosphate depletion is associated with increased intestinal absorption of calcium. A relevant finding is that phosphate depletion may enhance renal conversion of 25-hydroxy-cholecalciferol or 25-hydroxyvitamin D3 (25[OH]D3) into 1,25(OH)2D3, which stimulates intestinal absorption of calcium. Both metabolic and renal tubular acidosis are associated with an increased urinary excretion of calcium.
URINARY EXCRETION OF PHOSPHORUS
About 85% of serum inorganic phosphorus is filtered, and the ratio of H2PO4– to HPO42- depends on the pH. The proximal convoluted and straight tubules are the major sites of phosphorus reabsorption. According to micropuncture studies, when glomerular filtrate reaches the late proximal tubule, 70% of filtered phosphorus is reabsorbed. No phosphate is reabsorbed in the loop of Henle. Evidence was obtained that 10% of the filtered load may be absorbed beyond the early DCT. The tubular reabsorption of phosphate is a saturable process and displays a tubular maximum (Tm) reabsorptive capacity.
Phosphorus enters the brush-border membrane of the proximal tubule via the NaPi cotransport against a steep electrochemical gradient. This is energized by the sodium gradient generated by the basolateral sodium pump. Phosphorus moves out of the cell at the basolateral membrane mostly by a sodium-dependent transport (70%) and partly (30%) by a sodium-independent anion exchange system. Only the luminal cotransport is controlled by hormonal and other regulatory factors (e.g., PTH).
Sodium-Phosphate Cotransporters
The structure of a class of NaPi cotransporters in the brush-border membrane of the proximal tubule that facilitates the uptake of both sodium and phosphate in this segment of the nephron has been studied extensively. Many of the changes in the efficiency of phosphate transport are brought about by changes in the amount and activity of the NaPi cotransporters in this segment of the nephron.
Three families of NaPi cotransporters have been identified at the molecular level, named types I, II, and III NaPi cotransporters. Despite functional similarity, there is very little identity among them. Types I and II were cloned from rat and human renal mRNA by expression cloning strategy, whereas type III cotransporters were first identified on the basis of their function as viral cell surface receptors. PiT-1 cell surface receptor for gibbon ape leukemia virus, Glvr-1, and, PiT-2 cell surface receptor for rat amphotropic virus, RAM-1. In this regard, they are similar to CD4 that is a surface receptor for HIV. Types I and II cotransporters are expressed predominantly in the kidney and localized to the brush-border membrane of the proximal tubular cells.
Type 1 cotransporter was the first member to be cloned from a rabbit’s kidney. Its physiologic role, however, has not been well delineated. It is both a NaPi cotransporter and a chloride channel. As opposed to type II, type I produces electrogenic transport only at high concentrations of extracellular phosphorus concentrations. Studies suggest that type I functions as channel permeable not only to phosphorus and chloride but also to organic anions.
Type III is ubiquitously expressed and was initially assigned to fulfill a function of housekeeping NaPi cotransporters with no role as transcellular transporters. They have been assigned the role of supplying the basic cellular metabolic needs for phosphorus and as such were assumed to be located basolaterally in the kidney. The deeply ingrained notion that type III plays no role in renal transtubular vectorial transport was recently reassessed. New experimental data advanced evidence that PiT-2, a member of type III, which is localized to the brush-border membrane in proximal tubule epithelia, is a novel mediator of phosphorus reabsorption in the proximal tubule and is regulated by dietary phosphorus (30).
Type II cotransporters are the most abundant of the transporters and are also the major target for regulation by metabolic and hormonal factors including PTH, phosphatonins such as fibroblast growth factor 23 (FGF23), vitamin D, and dietary phosphate. Type II is largely responsible for renal phosphate reabsorption, as indicated by knockout experiments. Targeted inactivation of type II (Npt2) in mice leads to severe phosphate wasting, 70% to 85% reduction in phosphate reabsorption, hypercalciuria, and skeletal abnormalities. It has been generally assumed that NaPiIIc accounts for the remaining 15% to 30% of phosphorus transport capacity. This view has been questioned by recent experiments that suggest that Npt2c in mice do not play a role in phosphorus transport. Npt2c knockout mice do not develop phosphaturia or hypophosphatemia. The Npt2c knockout mice exhibited hypercalcemia, hypercalciuria, elevated plasma 1,25(OH)2D3, and no bone disease (31).
Three isoforms of rat type IIa cotransport have been described (32, 33). They appear to be products of alternative splicing, and are designated NaPiIIα, NaPiIIβ, and NaPilly. These isoforms are expressed as proteins at the brush-border membrane. None of the three spliced isoforms induced expression of NaPi transporter when injected into oocytes. However, when NaPiIIy cRNA was coinjected with the type IIa transporter into oocytes, it completely abolished the transport function of the type IIa cotransporter. Thus, alternative splicing may exert a great impact on phosphate transport in the proximal convoluted tubule. The physiologic impact of the aforementioned finding remains to be determined.
Type II cotransporters mediate both electrogenic divalent phosphate transport of HPO4–/3Na+ by NaPiIIa, and electroneutral divalent phosphate transport HPO4–/2Na+ by NaPiIIc. Type III cotransporters mediate monovalent phosphate electrogenic transport of H2PO4–/2Na+ by PiT-2. NapiIIa exhibits the fastest response to dietary phosphorus.
The function of NaPiIIa is closely linked to Na+/H+ exchanger regulating factor-1 (NHERF1), a membrane scaffold protein that plays a crucial role in binding to and anchoring NaPIIIa to the apical membrane. Phosphorylation of NHERF1 by PTH leads to reduced binding of NaPiIIa followed by its endocytosis, internalization, and degradation. This results in decreased phosphorus absorption and increased urinary excretion.
By contrast to rodents, where NaPiIIa has been the principal player in phosphorus absorption, in humans its role is less apparent. There are indications that NaPiIIc may be the dominant phosphorus transporter in humans. Loss-of-function mutation of the NaPiIIc gene is associated with excessive urinary wasting of phosphorus in patients with hereditary hypophosphatemic rickets with hypercalciuria, a human phenotype similar to that of NaPiIIa gene knockout rodents. Mutation of NaPiIIa as a cause of hypophosphatemia in humans has not been reported yet. The above observations underscore the importance of species differences in the regulation of physiologic process.
The essential role of NaPiIIa in tubular phosphorus in vivo has been challenged when exploring the response to intravenous bolus injection of PTH in parathyroidectomized rats. As expected, the phosphaturic effect of PTH was associated with a striking downregulation of NaPiIIa protein expression in brush-border membrane. However, a complete recovery of tubular phosphorus reabsorption that followed was not associated with a membrane recovery of the phosphorus transporter, suggesting that other transport mechanisms may fully compensate for the lack of NaPiIIa (34).
Dietary and Metabolic Factors
Urinary excretion of phosphorus depends on oral phosphorus intake to a great extent. Increased dietary phosphorus is associated with increased total and fractional urinary excretion of phosphorus. This may occur even in the absence of detectable changes in the serum level and filtered load of phosphorus. The state of parathyroid activity seems to play an important role in this phosphaturic response to phosphate load; in fact, this response has been used as a diagnostic test for hyperparathyroidism. Oral intake of 3 g of elemental phosphorus has been reported to increase the excretion of phosphorus to the maximum of 35% of the filtered load in normoparathryoid subjects, but the fractional phosphate excretion exceeds 35% in hyperparathyroid patients. However, although the presence of parathyroid hyperactivity intensifies with phosphaturic response to a phosphate load, the phosphaturic response may be observed in hypoparathyroid patients as well.
Phosphate depletion resulting from phosphate-deficient diets or intestinal phosphate losses is associated with a decrease in urinary excretion of phosphate to negligible amounts. This avid reabsorption of phosphorus is reversed by fasting and acidosis. Animal experiments suggest that increased insulin secretion during phosphorus deprivation contributes to the decreased urinary excretion of phosphorus in the urine.
Animal experiments demonstrated that a low phosphate diet increases the apical expression of type II cotransporters. Metabolic acidosis increases urinary phosphate excretion at the level of brush-border membranes,
and NaPiII abundance is reduced. Animal experiments demonstrated that growth hormone, thyroid hormone, insulin, and insulin-like growth factor increase phosphorus reabsorption and upregulate NaPiIIa expression.
and NaPiII abundance is reduced. Animal experiments demonstrated that growth hormone, thyroid hormone, insulin, and insulin-like growth factor increase phosphorus reabsorption and upregulate NaPiIIa expression.
Acute expansion of ECF volume with intravenous saline increases the urinary excretion of phosphorus; conversely, acute depletion of ECF volume tends to decrease urinary phosphorus (35). However, the effect of chronically increased oral intake of sodium chloride on urinary phosphorus excretion and phosphorus balance is unknown. In this regard, patients with primary hyperaldosteronism showed no changes in urinary phosphorus excretion but exhibited hypercalciuria.
A high oral intake of calcium is associated with a decreased urinary excretion of phosphorus. Two factors may account for this observation. First, calcium may depress intestinal absorption of phosphorus by forming nonabsorbable complexes with phosphorus. Second, large amounts of oral calcium may suppress the secretion of PTH and reduce urinary excretion of phosphorus. In contrast to its effect when given by the oral route, an intravenous load of calcium produces an acute increase in serum phosphorus concentration and augments excretion of phosphorus in the urine (2). The rise in serum phosphorus has been attributed to a direct effect of hypercalcemia, namely, promotion of the release of intracellular phosphorus into the circulation (2). This transient phosphaturia is followed by a substantial fall in urinary phosphorus excretion owing to suppression of parathyroid activity (7). In addition, hypercalcemia may exert a direct effect on the kidney, enhancing tubular reabsorption of phosphorus independent of parathyroid activity (36). This effect may be mediated by CaSR stimulation in the proximal tube (37). In contrast to this observation, however, is the finding that restoration of normocalcemia with intravenous calcium in patients with hypoparathyroidism is associated with increased urinary excretion of phosphorus. Likewise, the enhanced excretion of phosphorus that follows the administration of vitamin D to patients with hypoparathyroidism may be at least partly attributable to the restoration of the serum calcium level to normal. A recent study demonstrated that vitamin D downregulates NaPiIIa abundance in parathyroidectomized rats (38).
Acute loads of phosphorus in parathyroidectomized animals produce a net decrease in tubular reabsorption of phosphorus despite a markedly increased filtered load. This change has been linked with the attendant fall in serum calcium concentration and indeed may be reversed by maintaining a constant calcium level (39). This and the foregoing observations show the dependence of renal handling of phosphorus on serum levels of calcium and emphasize the complexity of their interrelationship. A rich phosphate diet in experimental animals downregulates the apical tubular expression of the NaPiII protein.
States of rapid catabolism with increased destruction of body tissues and metabolic acidosis are associated with hyperphosphatemia and phosphaturia. Similarly, cytolysis associated with the administration of cytotoxic agents to patients with neoplasms, especially neoplasms of lymphatic origin, is followed by severe hyperphosphatemia, phosphaturia, and hypocalcemia. Conversely, rapid regrowth of lymphatic tumors may lead to hypophosphatemia of marked degree because of incorporation of phosphorus in the tumor (40).
Intravenous administration of glucose has a dual effect on phosphorus metabolism. First, intravenous glucose tends to lower serum phosphorus, probably by incorporating phosphorus into the intracellular pool during the process of glucose phosphorylation. Second, glucose appears to have a direct renal effect in that it suppresses the reabsorption and increases the urinary excretion of phosphate. The competition between glucose and phosphate for transport across the epithelium of the proximal tubule has been demonstrated in studies with isolated renal tubules (41). This competition may be most important in states of massive glucosuria with uncontrolled diabetes mellitus.
Most diuretic agents acutely increase urinary phosphorus excretion. However, with the development of ECF volume depletion, the phosphaturic response of diuretics is blunted and may be restored with replacement of urinary losses of sodium and water. Neither the phosphaturic effect of thiazide nor that of acetazolamide seems to be dependent on the presence of parathyroid glands; however, the phosphaturic effect of these diuretics is linked to their ability to inhibit the enzyme carbonic anhydrase. Acidosis increases and alkalosis reduces urinary excretion of phosphorus.
Denervation of kidneys leads to an increase in urinary excretion of phosphorus because of an increased production of dopamine and decreased α– and β-adrenergic renal receptor activity. This denervation-related phosphaturia may contribute to renal losses of phosphorus after kidney transplantation.
Recent experiments in intact and parathyroidectomized rats demonstrated a rapid phosphaturic response to duodenal load of phosphate. Furthermore, protein extracts from homogenates of small intestine that were infused into animals elicited a phosphaturic response. As per suggestions based on the aforementioned observations, the intestine has luminal “sensors of phosphate” that sense increased luminal phosphate concentration and release a substance into the circulation that inhibits renal phosphate reabsorption. The nature of this substance remains to be defined (42).
Regulation of Serum Calcium and Phosphorus Concentration by Hormonal Factors Vitamin D and Its Metabolites
The term “vitamin D” was first introduced by McCollum in 1922 for the antirachitic factor isolated from cod liver oil (43). There are two naturally occurring sterol precursors of vitamin D, namely, ergosterol, which is present in plants, and 7-dehydrocholesterol, which is found in animals and humans. Under exposure to ultraviolet irradiation, ergosterol is converted into ergocalciferol (calciferol), which is known as vitamin D2. Vitamin D1 is not one compound but a mixture of many sterols with antirachitic activity.
The main source of vitamin D in humans is endogenous vitamin D3, produced by ultraviolet irradiation of 7-dehydrocholesterol in the skin. Areas of skin in most adults contain 3% to 4% of 7-dehydrocholesterol, which is located beneath the stratum corneum. Therefore, excessive amounts of pigment in the skin may interfere with the production of vitamin D3. The cutaneous synthesis of vitamin D3 is quite complex. Previtamin D3 is formed from its precursor 7-dehydrocholesterol. The preceding conversion depends on the levels of 7-dehydrocholesterol and is mediated by initial exposure to ultraviolet light. However, prolonged exposure to ultraviolet light may inactivate previtamin D3 and transform it to the inert photoproducts, lumisterol and tachysterol. The level of 7-dehydrocholesterol decline with age; therefore, older age predisposes to vitamin D deficiency. Vitamin D3, also known as cholecalciferol, is formed from previtamin D3 by thermal isomerization of 2 to 3 days in the skin and also is rapidly degraded by sunlight. Therefore, excessive exposure to sunlight cannot cause vitamin D intoxication because sunlight destroys any excess of vitamin D3 produced in the skin. Ten to fifteen minutes of exposure to sunlight can provide sufficient amounts of vitamin D3 for several days’ consumption.
The main source of exogenous vitamin D in the United States is milk, which contains about 400 units of vitamin D2 in each quart. The daily requirement of vitamin D in infants is about 400 units; in older age groups, the requirement is lower, as low as 70 units/day. This modest estimate has been recently challenged because of the high frequency of vitamin D deficiency in the adult and elderly population. Accordingly, higher intake of vitamin D in the range of 600 to 800 units/day has been recommended by some investigators (44).
METABOLISM OF VITAMIN D
Cholecalciferol is metabolized in the liver into 25(OH)D3, which has a more potent antirachitic activity in vivo than the parent compound, vitamin D undergoes 25-hydroxylation in the liver by 25(OH)ase. It has been generally accepted that 25(OH)ase is not a tightly regulated enzyme, but its activity is reduced by 50% in animals receiving vitamin D. However, DeLuca et al. demonstrated inhibition of hepatic production of 25(OH)D3 by 1,25(OH)2D3 in rats, thus suggesting feedback control (45). A decrease in the level of 25(OH)D3 in patients consuming anticonvulsive drugs, such as phenobarbital, phenytoin, primidone, carbamazepine, and rifampin, has been attributed to the induction of cytochrome P-450 enzymes, which leads to increased turnover of vitamin D, including catabolism resulting in vitamin D deficiency and bone disease (46). After enterohepatic circulation, 25(OH)D3 is further metabolized in the kidney into 1,25(OH)2D3, which is the most active metabolite of vitamin D. On a weight basis, it is 10 times more effective than vitamin D3 in curing rickets and 100 times more potent than 25(OH)D3 in stimulating calcium mobilization from the bone. When plasma calcium and phosphate levels are normal, 25(OH)D-1α(OH)ase activity in the kidney is reduced, and instead 25(OH)D-24(OH)ase activity prevails and metabolizes 25(OH)D3 into 24,25(OH)2D3. Calcitriol is an important negative regulator of itself. It exerts a feedback inhibition of 25(OH)D-1α(OH)ase. Current evidence indicates that this inhibition does not reflect a direct action of 1,25(OH)2D3 on 25(OH)D-1α(OH)ase gene promoter but rather it is an indirect effect. 1,25(OH)2D3 appears to inhibit the known PTH-induced activation of 25(OH)D-1a(OH)ase gene promoter via cyclic-AMP (cAMP). It is the PTH-generated cAMP that induces directly the gene promoter of 25(OH)D-1α(OH)ase resulting in increased production of 1,25(OH)2D3 from 25(OH) D3 (47). In this regard, previous experiments advanced evidence that vitamin D blocks the formation of cAMP by PTH in the kidney, both in vivo and vitro. The aforementioned findings shed light on the mechanism by which 1,25(OH)2D3 suppresses the activation of 25(OH)-1α(OH)ase (48).
It has been shown that 25(OH)D3 in complex with its carrier protein, the vitamin D-binding protein, is filtered through the glomerulus and reabsorbed in the proximal tubules by the endocytic receptor megalin. Endocytosis is required to preserve 1,25(OH)2D3 and deliver it to the cells as the precursor for the generation
of 1,25(OH)2D3. These findings contradict the previously held view that 1,25(OH)D3 was free, and as such diffused from the circulation across the basolateral surface of proximal tubular cells into the cytosol, to be converted by 25(OH)D-1α(OH)ase to 1,25(OH)2D3. Megalin knockout mice (megalin-1-mice) are unavailable to retrieve the 25(OH)D3 from the glomerular filtrate and develop vitamin D-deficiency state and bone disease. Such a role of the tubular reabsorption process is suggested by observations in patients with renal tubular defects. Similar to megalin knockout mice, patients who suffer from Fanconi syndrome are unable to reabsorb filtered macromolecules and exhibit vitamin D deficiency and bone disease (rickets and osteomalacia). Furthermore, it has been shown in patients with different degrees of renal failure that the GFR was directly correlated with plasma concentrations of 1,25(OH)2D3, suggesting that glomerular filtration is one of the determinants of 1,25(OH)2D3 synthesis by kidney (49).
of 1,25(OH)2D3. These findings contradict the previously held view that 1,25(OH)D3 was free, and as such diffused from the circulation across the basolateral surface of proximal tubular cells into the cytosol, to be converted by 25(OH)D-1α(OH)ase to 1,25(OH)2D3. Megalin knockout mice (megalin-1-mice) are unavailable to retrieve the 25(OH)D3 from the glomerular filtrate and develop vitamin D-deficiency state and bone disease. Such a role of the tubular reabsorption process is suggested by observations in patients with renal tubular defects. Similar to megalin knockout mice, patients who suffer from Fanconi syndrome are unable to reabsorb filtered macromolecules and exhibit vitamin D deficiency and bone disease (rickets and osteomalacia). Furthermore, it has been shown in patients with different degrees of renal failure that the GFR was directly correlated with plasma concentrations of 1,25(OH)2D3, suggesting that glomerular filtration is one of the determinants of 1,25(OH)2D3 synthesis by kidney (49).
PTH seems to act as a tropic hormone in stimulating the production of 1,25(OH)2D3 in the kidney. Thus, with intact parathyroid glands, changes in serum calcium indirectly regulate renal production of 1,25(OH)2D3 by altering the secretion of PTH. Specifically, hypocalcemia stimulates and hypercalcemic inhibits the synthesis of 1,25(OH)2D3. In addition, there is evidence that calcium acts directly to alter renal synthesis of calcitriol. Low serum phosphorus stimulates and high serum phosphorus suppresses the renal synthesis of 1,25(OH)2D3 independent of PTH. Several other factors control the formation of 1,25(OH)2D3. The novel group of humoral phosphaturic factors, termed “phosphatonins,” “FGF23,” and others, decrease 1,25(OH)2D3 by inhibiting the activity of 25(OH)d-1α(OH)ase. Furthermore, FGF23 synthesis is enhanced by 1,25(OH)2D3. It has been proposed that FGF23 is a negative feedback regulator of 25(OH)d-1α(OH)ase.
Growth hormone via increased synthesis of insulin-like growth factor I stimulates the activity of 25(OH) d-1α(OH)ase. Chronic metabolic acidosis in humans increases the serum levels of calcitriol (50). This effect could be mediated by acidosis-induced urinary losses of phosphate, leading to cellular phosphate depletion. Once it is formed, 1,25(OH)2D3 is metabolized to several less active metabolites in target tissues (13, 51). These transformations are enhanced by the hormone itself and thus may serve to decrease the biologic activity of the hormone once it has carried out its biologic functions. In addition, 1,25(OH)2D3 is excreted in bile as a monoglucuronide, other polar metabolites, and a 23-carbon acid, calcitroic acid (11, 13, 52). 1,25(OH)2D3 undergoes enterohepatic circulation in humans and various animal species. Proximal tubular cells are the major site of calcitriol formation. In addition, calcitriol may be produced in decidual cells, keratinocytes, bone cells, endothelial cells, peripheral monocytes, parathyroids, colon, prostate, breast and activated macrophages, where it may also exert a local autocrine or paracrine effect. The main aspects of the metabolism of vitamin D are shown in Figure 6-2.
Dihydrotachysterol (DHT3) is an analog of vitamin D used in the treatment of hypoparathyroidism. At high doses, it is more effective than vitamin D in mobilizing calcium from the bone, but in low doses it is less effective in curing rickets. DHT3 undergoes hydroxylation in the liver to 25(OH)DHT3, which is the active form of DHT3. Thus, DHT3 does not require the presence of the kidneys for the synthesis of its active metabolite. 1α-Hydroxycholecalciferol (1α[OH]D3) is a synthetic sterol that undergoes 25-hydroxylation in the liver to 1,25(OH)2D3 and, like DHT3, does not require the presence of renal tissue for its conversion into the active form of vitamin D3. Calcitriol stimulates the metabolic clearance of 25(OH)D3. Increased calcitriol formation with increased serum concentration leads to a fall in the serum concentration of 25(OH)D3 (53).
EFFECT OF VITAMIN D ON INTESTINAL ABSORPTION
Vitamin D3 stimulates intestinal absorption of calcium and phosphorus. The effect of vitamin D on calcium absorption becomes measurable several hours after its administration and is blocked by actinomycin D. Circulating calcitriol is a major regulator of intestinal calcium absorption. It exerts its effect mainly by a genomic mechanism mediated by binding to cytosolic vitamin D receptors (VDRs). The calcitriol-VDR structure complexes with retinoic X receptor (RXR) in the target cell nucleus, interacts with specific DNA sequences of calcitriol-responsive genes, and modulates transcriptional and posttranscriptional synthetic pathways. 1,25(OH)2D3 complex with its specific cytosolic VDR promotes mucosal epithelial calcium uptake by induction of the apical calcium channel TRPV6. This is the rate-limiting step in calcium transport. 1,25(OH)2D3 produces a cytosolic calcium-binding protein calbindin D that facilitates transcellular calcium movement, and it upregulates the basolateral PMCA1 that pumps calcium out of the cell.
The promotion of intestinal calcium absorption by vitamin D, at least partly, may be a rapid nongenomic response; the putative membrane receptors for 1,25(OH)2D3 may mediate this process. The active extrusion of calcium at the basolateral side by plasma membrane-bound Ca2+ ATPase operates also in the absence of vitamin D. However, 1,25(OH)2D3 has been shown conclusively to stimulate the activity and promote the
synthesis of the plasma membrane calcium pump. The increased synthesis of calcium-binding protein within the intestinal cell and the synthesis of an increased number of calcium pump units enhance the extrusion of calcium from within the intestinal cell into the ECF space.
synthesis of the plasma membrane calcium pump. The increased synthesis of calcium-binding protein within the intestinal cell and the synthesis of an increased number of calcium pump units enhance the extrusion of calcium from within the intestinal cell into the ECF space.
![]() Figure 6-2 Metabolism of vitamin D. The major source of vitamin D3 is its production in the skin; the other important source is diet. PTH, parathyroid hormone. |
EFFECT OF VITAMIN D ON BONE METABOLISM
Vitamin D promotes mineralization of the organic bone matrix. This action appears to be at least partly secondary to the effect of vitamin D on enhancing the intestinal absorption of calcium and phosphorus and thus maintaining their normal ECF concentrations. Some evidence supports a direct role of vitamin D in bone accretion (54). However, it has shown that vitamin D-deficient osteomalacia may be cured with the intravenous administration of calcium and phosphorus despite the persistence of a vitamin D-deficiency state (55).
Evidence suggests that vitamin D and its metabolites 25(OH)D3 and 1,25(OH)2D3 mobilize calcium and phosphorus from bone, an effect that has been demonstrated both in vivo and vitro (56, 57). Therefore, this action
of vitamin D may increase serum calcium concentration independently of its enhancement of the intestinal transport of calcium. Studies in animals have shown that vitamin D stimulates both osteocytic and osteoclastic bone resorption, and this action does not require the presence of PTH (56). Calcitriol induces differentiation of monocytic cells into mature osteoclasts, and it increases the number of osteoclasts. 1,25(OH)2D3 interacts with VDRs in osteoblasts to induce the expression of the receptor activator of nuclear factor kappa B (NF-κB) ligand (RANKL). RANKL binds to RANK on the plasma membrane of preosteoclasts and transforms them into mature osteoclasts. Osteoclasts dissolve the bone and release calcium and phosphorus into the circulation. Calcitriol increases osteoblast size and increases the synthesis of alkaline phosphatase and the blood level of osteocalcin. Interestingly, in vitro studies suggest that 1,25(OH)D3, but not other metabolites of vitamin D, may inhibit bone collagen synthesis (57).
of vitamin D may increase serum calcium concentration independently of its enhancement of the intestinal transport of calcium. Studies in animals have shown that vitamin D stimulates both osteocytic and osteoclastic bone resorption, and this action does not require the presence of PTH (56). Calcitriol induces differentiation of monocytic cells into mature osteoclasts, and it increases the number of osteoclasts. 1,25(OH)2D3 interacts with VDRs in osteoblasts to induce the expression of the receptor activator of nuclear factor kappa B (NF-κB) ligand (RANKL). RANKL binds to RANK on the plasma membrane of preosteoclasts and transforms them into mature osteoclasts. Osteoclasts dissolve the bone and release calcium and phosphorus into the circulation. Calcitriol increases osteoblast size and increases the synthesis of alkaline phosphatase and the blood level of osteocalcin. Interestingly, in vitro studies suggest that 1,25(OH)D3, but not other metabolites of vitamin D, may inhibit bone collagen synthesis (57).
The exact role of 24,25(OH)D3 in mineral metabolism is unknown and controversial. Many investigators view it as an inactive waste product of vitamin D catabolism. In animals, the hormone is metabolized to 1,24,25(OH)D3 and becomes active in intestinal absorption of calcium. In normal, hypoparathyroid, and anephric humans, however, 24,25(OH)D3 acts directly to increase intestinal absorption of calcium, even when given in relatively low doses (58). This effect of 24,25(OH)D3 is associated with positive calcium balance without changes in serum concentration or urinary excretion. In view of this observation and the previously reported effect of 24,25(OH)2D3 to promote the synthesis of protein by chondrocytes, it has been proposed that 24,25,(OH)D3 may be the metabolite that is directly involved in a skeletal metabolism (58). Furthermore, it has been reported that 1α(OH)D3 alone does not prevent rickets in chicks, whereas 24,25(OH)2D3 alone is effective (59). Experimental studies also have demonstrated that 24,25(OH)2D3 may play an important role in the suppression of bone resorption in rats after nephrectomy (60). In vitro studies demonstrated that 24,25(OH)D3 antagonizes the osseous calcium-mobilizing effect of calcitriol (61).
Recent experiments from our laboratory showed that 1,25(OH)2D3 induced a dose-dependent increase in calcium efflux from cultured bone. This increase was completely obliterated by inhibition of protein kinase C (PKC) with either staurosporine or cephalostin c. In cultured rat calvariae, 1,25(OH)2D3 also induced a dose-dependent translocation of PKC from cytosol to membrane. This activation of PKC by 1,25(OH)2D3 occurred following 30 seconds of incubation, peaked at 1 minute, and disappeared by 5 minutes. 1,25(OH)2D3 did not increase cAMP production in similarly cultured calvaria. These results suggest that the action of 1,25(OH)2D3 on calcium flux from bone tissue is mediated by the activation of PKC (62).
Similarly, we have shown in the same experimental model that 24,25(OH)D2D3 induced a dose-dependent flux of calcium into the bone. This effect was mediated by an inactivation of PKC. Thus, the action of 1,25(OH)2D3, which mobilizes calcium from bone, and 24,25(OH)2D3, which inhibits bone resorption, are mediated by activation and inhibition of PKC, respectively (63). It is interesting that other investigators demonstrated that 1,25(OH)2D3 activates PKC, rapidly increases intracellular calcium, and stimulates polyphosphoinositide hydrolysis in colonic epithelia (64). Thus, there is mounting evidence demonstrating a role of PKC activation in mediating the nongenomic effects of vitamin D.
EFFECT OF VITAMIN D ON RENAL HANDLING OF PHOSPHORUS AND CALCIUM
The effect of vitamin D on renal handling of phosphorus has been the subject of numerous investigations. The main difficulty encountered in interpreting the changes in urinary excretion of phosphorus has been related to the calcemic actions of vitamin D, which, by suppressing PTH secretion, indirectly alter renal handling of phosphorus. Consequently, the enhanced tubular reabsorption of phosphorus following the administration of vitamin D to patients with osteomalacia and rachitic animals with intact parathyroid glands could be accounted for either by inhibition of PTH secretion or a direct tubular action of vitamin D. The results of studies in animals suggest that both 25(OH)D3 and 1,25(OH)2D3 acutely enhance tubular reabsorption of phosphorus (65); in rats, this effect requires the presence of either endogenous or exogenous PTH. The antiphosphaturic effect on minimal doses of 1,25(OH)2D3 was demonstrated in chronic studies in vitamin D-deficient rats; this effect was reported to be associated with upregulated NaPiII expression. The effects of 1,25(OH)2D3 are summarized in Figure 6-3.
Recent studies from our laboratory addressed the mechanism(s) underlying the effects of vitamin D on renal handling of phosphate both in acute experiments, using opossum kidney (OK) cell line, and in chronic experiments using metabolic clearances in parathyroidectomized rats infused with 1,25(OH)2D3 without and with PTH via osmotic minipumps over 7 days. The acute studies in OK cells reproduced our previous results in rats (65, 66). These experiments demonstrated that treatment for 24 hours with 1,25(OH)2D3 antagonized the effect of PTH to inhibit phosphate uptake in OK cells. This action of 1,25(OH)2D3 was associated with
suppressed PTH-induced activation of the second messenger transduction pathway, the adenylate cyclase/cAMP/PKA system. Interestingly, this response to vitamin D was accompanied by a substantial diminution in the expression of the PTH/PTH-related peptide (PTHrP) receptor. The latter could at least partly account for the inhibition of adenylate cyclase-cAMP activation by PTH. Likewise, it is interesting that the observed PTH-triggered rise in intracellular calcium, which presumably resulted from PTH-induced activation of the second signal transduction pathway, namely the activation of PKC, was not altered by 1,25(OH)2D3. In contrast to the acute studies, in chronic experiments, 1,25(OH)2D3 enhanced the phosphaturic effect of PTH despite retaining the concomitant reduction in urinary cAMP excretion. We observed that both NapIII mRNA and NaPiII protein were significantly reduced when 1,25(OH)2D3 was infused alone, when PTH was given alone, and, most strikingly, when both were given together. Furthermore, the observed vitamin D-induced downregulation of the PTH/PTHrP receptor was reversed in the chronic study when PTH and 1,25(OH)2D3 were given together. The latter could contribute at least partly to the enhancement of PTH-induced phosphaturia by 1,25(OH)2D3. Thus, as opposed to acute conditions where vitamin D blunts the phosphaturic effect of PTH, chronic administration of vitamin D exerts the opposite effect (67). Therefore, it is apparent that the effect of vitamin D on renal handling of phosphate is very complex and depends on many variables.
suppressed PTH-induced activation of the second messenger transduction pathway, the adenylate cyclase/cAMP/PKA system. Interestingly, this response to vitamin D was accompanied by a substantial diminution in the expression of the PTH/PTH-related peptide (PTHrP) receptor. The latter could at least partly account for the inhibition of adenylate cyclase-cAMP activation by PTH. Likewise, it is interesting that the observed PTH-triggered rise in intracellular calcium, which presumably resulted from PTH-induced activation of the second signal transduction pathway, namely the activation of PKC, was not altered by 1,25(OH)2D3. In contrast to the acute studies, in chronic experiments, 1,25(OH)2D3 enhanced the phosphaturic effect of PTH despite retaining the concomitant reduction in urinary cAMP excretion. We observed that both NapIII mRNA and NaPiII protein were significantly reduced when 1,25(OH)2D3 was infused alone, when PTH was given alone, and, most strikingly, when both were given together. Furthermore, the observed vitamin D-induced downregulation of the PTH/PTHrP receptor was reversed in the chronic study when PTH and 1,25(OH)2D3 were given together. The latter could contribute at least partly to the enhancement of PTH-induced phosphaturia by 1,25(OH)2D3. Thus, as opposed to acute conditions where vitamin D blunts the phosphaturic effect of PTH, chronic administration of vitamin D exerts the opposite effect (67). Therefore, it is apparent that the effect of vitamin D on renal handling of phosphate is very complex and depends on many variables.
Large doses of vitamin D cause hypercalciuria, possibly by increasing absorption of calcium from the intestine. In contrast, acute clearance studies in dogs showed an increased renal tubular absorption of calcium after intravenous administration of vitamin D (68, 69). Vitamin D, however, does not appear to be essential for the renal conservation of calcium, because urinary calcium excretion may be reduced to extremely low levels in osteomalacia resulting from vitamin D deficiency (55).
In addition to the effect of vitamin D on the intestine, bone, and kidney, vitamin D acts directly on parathyroid tissue to suppress secretion of PTH. Studies in rats in our laboratory demonstrated that physiologic
amounts of 1,25(OH)D3 inhibit the levels of PTH mRNA, independent of serum calcium levels (70). This action of calcitriol is mediated by the VDR. Calcitriol acts on at least two negative regulatory elements upstream in the 5′ flanking region of the PTH gene to suppress transcription. Furthermore, calcitriol modulates secretion and synthesis of PTH by increasing gene expression of the VDR in the parathyroid gland (53).
amounts of 1,25(OH)D3 inhibit the levels of PTH mRNA, independent of serum calcium levels (70). This action of calcitriol is mediated by the VDR. Calcitriol acts on at least two negative regulatory elements upstream in the 5′ flanking region of the PTH gene to suppress transcription. Furthermore, calcitriol modulates secretion and synthesis of PTH by increasing gene expression of the VDR in the parathyroid gland (53).
In view of this information, a feedback loop may be formulated that has the following sequence: PTH stimulates the formation of 1,25(OH)2D3, and 1,25(OH)D3 closes the negative feedback loop by suppressing the secretion of PTH. Thus, among other functions, 1,25(OH)2D3 may have a modifying effect on the secretion of PTH. FGF23 inhibits renal synthesis of 1,25(OH)2D, and indirectly, via lowering the active vitamin D metabolite, increases PTH secretion. This effect has been observed in chronic kidney disease (CKD). This assumption is supported by the observation that the FGF23 rise in CKD precedes that of PTH and the decrease in 1,25(OH)2D3 (53).
Vitamin D activity in the serum and in other tissues may be measured by both bioassay and radioimmunoassay techniques. The radioreceptor assay can determine the serum levels of various metabolites. These competitive protein-binding assays have great potential importance in determining the mechanisms underlying clinical disorders secondary to abnormalities in vitamin D metabolism.
Parathyroid Hormone
PTH is a single-chain polypeptide of 84 amino acid residues (mol wt 9,500) with biologic activity in the N-terminal 1 to 34 region of the molecule. The biosynthesis of the hormone starts with prepro-PTH, a 110 amino acid chain polypeptide that is the translation product of PTH mRNA. Pro-PTH is produced after cleavage of 21 amino acids. PTH is produced after additional cleavage and stored in secretary droplets. The amount of stored hormones is sufficient for basal secretion over 5 to 6 hours and 2 hours of augmented secretion. Thus, the synthesis is closely linked to secretory activity.
PTH plays a central role in the physiologic regulation of serum calcium concentration. Serum calcium concentration is maintained within a very narrow range, primarily because of a feedback mechanism in which minimal changes in ionized calcium alter the secretory rate of PTH, which then restores the ionized calcium to its normal concentration by its action on bone. Serum concentration of phosphorus is not feedback regulated; therefore, it varies over a relatively wide range. Recent studies, however, suggest that changes in serum phosphate may be involved in the regulation of PTH secretion. There is a direct relationship between serum phosphate level and the secretion of PTH. Thus, high serum phosphate levels increase and low levels decrease the synthesis and secretion of PTH. This appears to be a posttranscriptional effect. This effect appears to be independent of changes in serum calcium and vitamin D (71, 72).
It is apparent that serum-ionized calcium is the single most important physiologic factor controlling the secretory rate of PTH. A sensitive inverse relationship has been demonstrated between ionized calcium and serum level of PTH (8). The parathyroid cells have a cell surface sensing mechanism to extracellular calcium concentration that also recognizes other divalent and polyvalent cations, such as magnesium and neomycin. This mechanism is based on a CaSR. The CaSR is a membrane of the G protein-coupled receptor family that responds to increased extracellular calcium by triggering the phospholipase C pathway and elevating inositol triphosphate, diacylglycerol, and intracellular calcium concentration. The increased intracellular calcium inhibits PTH secretion from parathyroid cells. The CaSR expressed in the parathyroid, thyroid, and kidney was cloned and characterized (23). Recently, another possible CaSR that is distinct from the G protein-coupled receptor was identified. This receptor (CAS) is a large protein known as gp330 megalin and is a member of the low-density lipoprotein receptor superfamily. It was identified in parathyroid tissue, but its role in parathyroid physiology is unknown. In addition to its acute effect on PTH secretion, chronic changes in serum-ionized calcium concentration, both hypercalcemia and hypocalcemia reduce or increase the steady-state level of PTH mRNA and synthesis of PTH. In vivo, calcitriol causes a 90% decrease in prepro-PTH mRNA at 48 hours; the effect starts after 2 hours. As opposed to calcium, which exerts both an acute and a chronic effect on PTH secretion, calcitriol does not have an acute effect on PTH secretion, but there is a decrease in PTH secretion after 12 to 24 hours (53).
An increase in PTH secretion also has been observed in cows during the administration of epinephrine, raising the possibility that the autonomic nervous system may play a role in controlling PTH secretion. An aberration in extracellular pH affects PTH secretion rate. A decrease in pH inhibits CaSR activity, leading to an increase in PTH secretion, whereas alkalinization enhances CaSR activity leading to a decrease in PTH secretion. In animal experiments, metabolic acidosis predisposes to elevated PTH levels with concomitant hypercalcemia, whereas alkalosis lowers PTH levels (73).
Peripheral Actions of Parathyroid Hormone and Parathyroid Hormone-Related Peptide
The peripheral actions of PTH and PTHrP on bone and kidney involve binding to cell surface receptors followed by activation of two pathways of signal transduction. Thus, PTH stimulates both the adenylate cAMP-PKA pathway and phospholipase C, which in turn leads to activation of PKC by diacylglycerol and an increase in intracellular calcium by inositol triphosphate. The stimulation of these two signaling pathways is mediated by coupling of the hormone-occupied receptor with two distinct G proteins, which link the receptor to effector pathways (74, 75). The gene for the human PTH receptor for bone and kidney has been cloned, sequenced, and expressed in African green monkey kidney (cos) cells. The evaluation of the structure and function relationship of the receptor and PTH is interesting. It has been demonstrated that the N-terminal fragment PTH sequence 1 to 34 reproduces all physiologic effects of PTH sequence 1 to 84. It has been shown that the amino acid sequences 10 to 15 and 24 to 34 of PTH are necessary for binding to the receptor. With regard to the biologic effects of PTH, it has been shown that the first two N-terminal amino acids 1 and 2 are required for the activation of adenylate cyclase-PKA pathway, whereas the amino acids sequence 28 to 34 are required for the activation of phospholipase C-PKC pathway. Indeed, the fragment PTH sequence 3 to 34 was shown in vitro to suppress phosphate transport without activation of adenylate cyclase-PKA pathway (74, 75, 76).
Another PTH receptor (type 2) that binds only PTH and does not bind PTH-related protein (PTHrP) has been found in the brain and intestines. The functions of this receptor are unknown.
EFFECT OF PARATHYROID HORMONE ON BONE
PTH plays a major role in bone remodeling. PTH increase bone turnover owing both to increase in osteoclast numbers and resorption and stimulation of bone formation by osteoblast activation.
Its receptors are expressed in bone-forming cells, osteoblasts, and proosteoblasts, but not in osteoclasts. Thus, although PTH acts to increase osteoclastic resorption, it appears that this effect is not mediated via receptors on osteoclasts but are indirect, occurring through the interaction of PTH with receptors on osteoblasts.
PTH-activated osteoblasts may enhance recruitment and stimulation of osteoclasts.
PTH augments release of mineral from bone by stimulating both osteocytic and osteoclastic bone resorption and possibly by enhancing calcium transport from the skeletal ECF into the systemic ECF. There is experimental evidence that the latter is a direct effect. The resulting increase in serum calcium concentration may be preceded by a short period of decreasing concentration because of an initial enhanced entry of calcium in bone cells.
The calcemic effect of PTH on bone requires the presence of vitamin D (77, 78). The impaired response to PTH in vitamin D deficiency may be because of either some permissive action of the vitamin or the mechanical blocking effect of the osteoid that coats the surface of the mineralized bone and thus prevents access of the PTH. Correction hypocalcemia per se in rats has been shown to restore the responsiveness of the bone to the action of the PTH in states of vitamin D deficiency. This observation is consistent with the possibility that calcium is a cofactor in the skeletal action of PTH. Recently, proposals hold that PTH acts on two distinct cellular systems in the bone: (a) the remodeling system and (b) the calcium mobilization or calcemic-homeostatic system. The remodeling system consists of osteoclasts that resorb old bone and osteoblasts that form new bone. In this system, bone resorption is balanced by bone formation; therefore, no mineral escapes into the circulation. The homeostatic system is based on the action of surface osteocytes and osteocytes occupying lacunar spaces that regulate the movement of calcium between the bone fluid and ECF. This mineral-releasing system is important in everyday regulation of serum calcium and requires 1,25(OH)2D3 in addition to PTH. Recent in vitro studies suggest that the calcium-mobilizing effect of PTH is mediated by activation of the phospholipase C-PKC signal transduction system (79).
EFFECT OF PARATHYROID HORMONE ON THE KIDNEY
The primary renal effect of PTH is to produce phosphaturia by depressing net phosphate reabsorption in the proximal tubule. This tubular effect involves PTH receptor-mediated intracellular formation of messenger cAMP, inositol triphosphate, diacylglycerol, and free cytosolic calcium and activation of PKA and PKC. These inhibit brush-border transport systems including NaPi cotransport and sodium-proton antiport exchange (74). The results of certain studies suggest additional effects of PTH in more distal parts of the nephron on
phosphorus absorption (80). Phosphate depletion produces resistance to the phosphaturic action of PTH in rats; however, this has not been shown yet in humans.
phosphorus absorption (80). Phosphate depletion produces resistance to the phosphaturic action of PTH in rats; however, this has not been shown yet in humans.
Type II Na/Pi cotransporters are believed to represent the major pathway of renal phosphate reabsorption and are the major target with respect to inhibition of proximal tubular reabsorption by PTH. Both acute and chronic administration of PTH downregulate the NaPiII protein in brush-border membrane of proximal tubular cells; however, only chronic administration downregulates NaPiII mRNA. In vivo and in vitro experiments showed that PTH causes retrieval of NaPiII cotransporters from the membrane. After internalization, they are routed to the lysosomes, where they are degraded.
It had been earlier assumed but never proven that NaPiII downregulated by PTH involves phosphorylation of the transporter by cAMP. Recent evidence suggests that PTH induces phosphorylation of the PDZI domain in NHERF1, a scaffold protein that binds to and anchors NaPiIIa to the apical membrane. Phosphorylation of the scaffold protein reduces its bindings to NaPiIIa and leads to NaPiIIa internalization and degradation in the lysosome (81).
Although the effect of PTH in the proximal tubule is to depress calcium reabsorption, its net effect is to decrease urinary calcium excretion in dogs, rats (82), and humans. The net increase in tubular reabsorption of calcium appears to be caused primarily by a distal action of the hormone, where approximately 10% to 20% of filtered calcium is reabsorbed (18). Thus, it appears that both the renal and the skeletal actions of PTH act jointly to increase serum concentrations of calcium. Because PTH may increase bicarbonate, sodium, and amino acid excretion, the hormone does not appear to increase the reabsorption of these substances in the distal nephron. Parathyroidectomy in rats is associated with downregulation of calcium channel TRPV5, calbindin D28K, and expression of basolateral calcium transporters. The exact molecular mechanism involved in PTH-induced increase in calcium transport in DCT and CNT has not been elucidated in detail yet. The classic concept that cAMP stimulates calcium reabsorption in this nephron segment has been questioned. Recent studies suggest that PTH stimulates calcium reabsorption in the distal nephron, independent of cAMP, via activation of the phospholipase C-PKC signal transduction pathway. Activation of PKC increases cell surface abundance of TRPV5 by inhibiting endocytosis. This mechanism of regulation of PKC may contribute to acute stimulation of TRPV5 and calcium absorption of PTH (83).
EFFECT OF PARATHYROID HORMONE ON INTESTINAL ABSORPTION OF CALCIUM
A role of PTH in the intestinal absorption of calcium has been suggested by several studies in both animals and humans. However, at present there is no evidence to support a direct action of PTH on calcium transport in the intestine. The fact that PTH enhances the conversion of 25(OH)D3 to 1,25(OH)2D3, which directly acts on the intestinal transport of calcium, may explain the apparent effects of the hormone. Even so, it is obvious that in states of vitamin D deficiency, elevated levels of circulating PTH fail to maintain normal absorption of calcium. Conversely, vitamin D may affect intestinal absorption in the absence of PTH in patients with hypoparathyroidism. The multiple actions of PTH are summarized in Figure 6-4.
RADIOIMMUNOASSAY OF PARATHYROID HORMONE
Radioimmunoassay for circulating PTH was introduced by Berson and Yalow (84) in 1963. Further studies led to the recognition of the heterogeneity of circulating PTH, which apparently represents various molecular species of the hormone. The glandular hormone (mol wt 9,500) consists of 84 amino acids (1-84 sequence) and has two terminals, amino (-NH2) and carboxy (-COOH). The circulating PTH consists of the glandular hormone and its fragments. At least two different molecular species of circulating PTH have been detected by different antisera, one with a molecular weight of 4,500 to 5,000 and one with a molecular weight of 7,000 to 7,500. Structurally, there are two major split products that can be characterized by their terminals. The first product has the N-terminal, is the biologically active fragment, and has an amino acid sequence 1 to 34. The second product has the C-terminal, is the biologically inactive fragment, and has an amino acid sequence 53 to 84. The level of total circulating immunoreactive PTH that reflects all molecular species appears to represent the chronic state of parathyroid function and is most useful in the diagnosis of parathyroid abnormalities. The level of the glandular (mol wt 9,500) species represents acute changes in parathyroid activity, such as those that occur after calcium infusion. Although it has been suggested that the level of the C-terminal fragment provides the best differentiation
between normal persons and patients with hyperparathyroidism, this seems paradoxical in view of the fact that the C-terminal is biologically inactive. It should be emphasized that radioimmunoassays that measure the C-terminal fragments do not provide a reliable estimate of parathyroid function in patients with renal insufficiency. This is because the clearance of C-terminal fragments is delayed in renal failure. Thus, the renal failure in radioimmunoassay of N-terminal species of PTH or the intact hormone molecule provides a better indication of parathyroid function. The intact PTH assay employs two antibodies, one binding the N-terminal and the second binding the C-terminal. One antibody is fixed on beads that are then exposed to serum. After incubation with the tested serum, the beads are separated and exposed to radiolabeled antibodies binding the opposite terminal.
between normal persons and patients with hyperparathyroidism, this seems paradoxical in view of the fact that the C-terminal is biologically inactive. It should be emphasized that radioimmunoassays that measure the C-terminal fragments do not provide a reliable estimate of parathyroid function in patients with renal insufficiency. This is because the clearance of C-terminal fragments is delayed in renal failure. Thus, the renal failure in radioimmunoassay of N-terminal species of PTH or the intact hormone molecule provides a better indication of parathyroid function. The intact PTH assay employs two antibodies, one binding the N-terminal and the second binding the C-terminal. One antibody is fixed on beads that are then exposed to serum. After incubation with the tested serum, the beads are separated and exposed to radiolabeled antibodies binding the opposite terminal.
Recently, it has been realized that current two-site assays designed to detect both an N-terminal and C-terminal epitopes of PTH may not be reliable. PTH molecules that are reactive in these assays are considered intact, but some have no bioactivity. For example, a loss of only six amino acids to yield PTH (83, 84, 85) eliminates all bioactivity but does not affect the immunoreactivity measured in most of these assays. In fact, about 50% of PTH detected in these assays in the serum of patients with chronic renal failure is not only biologically inactive but also exhibits an antagonistic effect on the biologic activity of 1 to 84 PTH. The newer immunodetection of PTH by whole PTH two-site assay that recognizes the first six amino acids appears to be more reliable in measuring only biologically active PTH.
Calcitonin
The discovery of calcitonin established the presence of a new regulatory system for calcium homeostasis.
Calcitonin is a polypeptide with 32 amino acid residues and was isolated from the parafollicular cells of the thyroid gland or ultimobranchial body in a wide variety of species. Hypercalcemia stimulates release of calcitonin, which tends to lower serum calcium concentration (85).
EFFECT OF CALCITONIN ON BONE
The major mechanism by which calcitonin lowers serum calcium and phosphorus concentrations is inhibition of bone resorption. This is associated with decreased osteoclastic activity and decreased urinary hydroxyproline excretion. In organ culture, after prolonged treatment with calcitonin, PTH may overcome the inhibitory effect of calcitonin and induce bone resorption. This phenomenon is termed “escape” and is also observed in vivo in animals with intact parathyroid glands chronically treated with calcium. An antagonism exists between calcitonin and glucocorticoid hormones, because glucocorticoids interfere with the hypocalcemic action of calcitonin.
The receptors of calcitonin have been cloned from human giant cell tumors of bone, human ovary, and breast cell line (75). The calcitonin receptor is expressed by osteoclasts, as opposed to PTH and calcitriol receptors, which are expressed only by osteoblasts. Similar to PTH receptors, calcitonin receptors couple to two signal transduction pathways, adenylate cyclase-PKA and phospholipase C-PKC via linking with G proteins. Calcitonin acts directly to inhibit osteoclast action on the bone and inhibits osteoclast motility in isolated osteoclast preparations.
EFFECT OF CALCITONIN ON THE KIDNEY
Calcitonin increases urinary excretion of phosphorus, sodium, potassium, and calcium. This effect is independent of PTH. In fact, calcitonin acts to reverse the effect of PTH in two organs. It inhibits bone resorption and increases urinary calcium excretion; both actions tend to lower serum calcium. The phosphaturic action of calcitonin has been found to be associated with an increased urinary excretion of cAMP.
Under normocalcemic conditions, calcitonin, and not PTH, has an important role in the maintenance of serum 1,25(OH)2D3 levels. Calcitonin enhances induction of 25(OH)D-1α(OH)ase transcription and protein expression. Because plasma calcitonin is increased during pregnancy and lactation, it has been proposed that this is the mechanism of increased 1,25(OH)2D3 observed during pregnancy and lactation when calcium requirements are increased (86). Likewise, it is important to remember that experiments conducted in thyroparathyroidectomized animals that are not replaced with calcitonin supplements may invalidate the results.
EFFECT OF CALCITONIN ON INTESTINAL ABSORPTION
The effect of calcitonin on intestinal absorption has not been studied extensively. Preliminary reports indicate, however, that calcitonin has no effect on intestine calcium absorption but may actively decrease the absorption of phosphorus, sodium, potassium, and chloride. The multiple actions of calcitonin are summarized in Figure 6-5.
The development of a sensitive radioimmunoassay for calcitonin has provided the means to study the control of secretion of this hormone. From a clinical standpoint, the radioimmunoassay serves as a valuable aid in the diagnosis of medullary carcinoma of thyroid, which is a calcitonin-secreting tumor.
Disorders of Calcium and Phosphorus Metabolism Associated with Hypocalcemia
VITAMIN D DEFICIENCY
Hypocalcemia is a common feature in vitamin D deficiency; however, this disorder may be present with a normal serum calcium concentration. For example, vitamin D-deficiency rickets in children evolves over three stages. During the first stage, serum calcium concentration is low, serum phosphorus is normal, and
immunoreactive PTH in the serum is normal (76, 87). There is no satisfactory explanation for the normal PTH in the presence of hypocalcemia. During the second stage, there is a rise in PTH activity, and serum calcium concentration rises to a normal level as serum phosphorus concentration decreases. In the third stage, which is the most severe, both serum calcium and phosphorus concentrations are low (78). It is unknown whether vitamin D-deficiency osteomalacia in adults shows a similar evolution.
immunoreactive PTH in the serum is normal (76, 87). There is no satisfactory explanation for the normal PTH in the presence of hypocalcemia. During the second stage, there is a rise in PTH activity, and serum calcium concentration rises to a normal level as serum phosphorus concentration decreases. In the third stage, which is the most severe, both serum calcium and phosphorus concentrations are low (78). It is unknown whether vitamin D-deficiency osteomalacia in adults shows a similar evolution.
Table 6-2 Common Causes of Vitamin D Deficiency | ||||||||||||||||||||||||||||||||||||
---|---|---|---|---|---|---|---|---|---|---|---|---|---|---|---|---|---|---|---|---|---|---|---|---|---|---|---|---|---|---|---|---|---|---|---|---|
|
The various common etiologies of vitamin D deficiency are listed in Table 6-2. Because vitamin D is fat-soluble, nutritional osteomalacia usually is associated with a deficient intake of food products containing fatty substances (78, 88). Partial gastrectomy may lead either to a simple dietary deficiency of vitamin D as a result of avoiding fatty foods or malabsorption of vitamin D. Small bowel disease may produce both malabsorption of vitamin D and mucosal resistance to its action. Bile salt deficiency interferes with vitamin D absorption, and hepatocellular failure may interfere with its metabolism. Factitious diarrhea caused by prolonged ingestion of laxatives also may cause vitamin D deficiency. Likewise, nephrotic syndrome is associated with urinary losses and low levels of circulating 25(OH)D3 (89).
In addition to vitamin D deficiency resulting from nutritional and GI causes, a group of disorders has been identified in which the deficiency is caused by an abnormal metabolism of vitamin D. Vitamin D-dependent rickets is an inherited autosomal recessive disorder. It appears during early infancy and responds to pharmacologic doses of vitamin D and physiologic doses of calcitrol. This disorder represents an inherited deficiency in the kidney enzyme 25(OH)2D3-1α(OH)ase, which converts 25(OH)2D3 into 1,25(OH)2D3 (88).
25(OH)D-1α(OH) is a mitochondrial cytochrome P-450 enzyme that functions in proximal tubular cells.
Although it is a key enzyme in vitamin D metabolism, its cloning was difficult because of the low level of gene expression. The recent cloning of the human 25(OH)D-1α(OH)ase deoxyribonucleic acid (DNA) and gene made it possible to screen for mutations. Vitamin D-dependent rickets type I (VDDR-1) occurs at high frequency in French Canadians; the disease locus in this population was mapped to chromosome 12q13-14 by linkage analysis. Renal 1α(OH)ase activity is regulated by PTH, calcitonin, calcium, phosphate, and 1,25(OH)2D3 itself. By contrast, the recently cloned 1α(OH)ase in macrophages is not stimulated by PTH or calcitonin; however, 8-Br-cAMP and interferon (IFN)-y increased the expression of the enzyme (90). FGF23 suppresses the renal enzyme 1α0(OH) hydroxylase. This is a key factor of secondary hyperparathyroidism of CKD at all stages. The level of FGF23 is elevated already in every stage of CKD (53).
Osteomalacia in patients ingesting phenobarbital is associated with low levels of circulating 25(OH) D3. It has been shown that the biologic half-life of vitamin D3 and 25(OH)D3 is shortened in phenobarbital-treated patients and that there is an accumulation of more polar metabolites, some of which are inactive (87). This rapid turnover and the production of inactive forms of vitamin D have been attributed to induction of microsomal enzyme activity in the liver. Phenytoin does not interfere directly with vitamin D metabolism but can induce hypocalcemia through reduced intestinal absorption of calcium and decreased release from bone. Low levels of circulating 25(OH)D3 also have been observed in patients with chronic hepatic failure (53, 88).
Dietary calcium deprivation per se in rats increases the clearance and inactivation of 25(OH)D3 and leads to vitamin D deficiency. It has been suggested that this form of vitamin D deficiency is caused by secondary hyperparathyroidism, which increases the renal production of calcitriol. The latter augments the degradation of 25(OH)D3 to inactive metabolites. Hypothetically, this mechanism may account for vitamin D deficiency observed in clinical states of calcium malabsorption, including GI diseases, resection, or bypass; chronic liver disease; anticonvulsant therapy; and morbid obesity (53, 91).
The clinical significance of abnormally low serum levels of 25(OH)2D3 in patients with nephrotic syndrome owing to excessive urinary losses of vitamin D-binding globulin has not been fully established yet. However, a study of bone histology in patients with nephrotic syndrome who had normal renal function revealed that the decrease in 25(OH)D3 level results in a decrease in ionized calcium, secondary hyperparathyroidism, and enhanced bone resorption as well as defective mineralization. Interestingly, vitamin D has receptors in glomerular foot processes. It plays a protective role in preserving integrity and function of podocytes and other glomerular elements, via its specific calcium channel.
Our unpublished observations suggest that vitamin D supplementation may alleviate nephrotic syndrome and reduce the degree of proteinuria. It is well known that proteinuria leads to loss of vitamin D and precipitates significant vitamin D deficiency. It may require huge amounts of vitamin D to achieve full normalization of its levels in serum. Vitamin D level measurement is mandatory to avoid overdose (89).
Understanding of the metabolic pathways of vitamin D may facilitate the investigation of various abnormalities.
For example, low levels of 1,25(OH)2D3 have been reported in patients with hypoparathyroidism. The lack of PTH and the presence of hyperphosphatemia may decrease the conversion of 25(OH)2D3 to 1,25(OH)2D3, which may explain the resistance to vitamin D in some patients with hypoparathyroidism. In support of this possibility is the finding of successful treatment of hypocalcemia with 1,25(OH)2D3 in patients with hypoparathyroidism.
HYPERPARATHYROIDISM
Hyperparathyroidism is a common cause of hypocalcemia. Hyperparathyroidism can cause hypocalcemia with paresthesias, muscle spasms (i.e., tetany), and seizures, especially when it occurs rapidly. Chronic hyperparathyroidism generally causes hypocalcemia so gradually that the only symptoms may be visual impairment from cataracts following years of hyperparathyroidism. Hyperparathyroidism may be secondary or idiopathic.
SECONDARY HYPERPARATHYROIDISM
Hyperparathyroidism may be caused by surgery. This variety of hyperparathyroidism may result from accidental removal of parathyroids or traumatic interruption of their blood supply. Very often, the parathyroid deficiency is transient in nature. Hypocalcemia that appears after excision of parathyroid adenoma results from functional suppression and hypofunction of the remaining normal glands. Hyperparathyroidism may be a component of multiple endocrine dysfunctions, including adrenal insufficiency owing to an autoimmune disorder. In hyperparathyroidism associated with pernicious anemia, an autoimmune mechanism also has been implicated. Autoimmune hyperparathyroidism is commonly a part of polyglandular autoimmune syndrome type I (APS-1), which is familial syndrome. It occurs during childhood and is inherited as an autosomal recessive trait caused by mutations in an autoimmune regulator gene (AIRE). Loss of immunologic tolerance to tissue-restricted antigens due to the absence of AIRE expression in the thymus leads to exit of autoreactive T cells from the thymus that trigger autoimmunity (92). Antibodies against IFN-ω and –α have been recently shown to be a sensitive and specific marker for APS-1. APS-1 is associated with mucocutaneous candidiasis, vitiligo, and adrenal insufficiency. Antibodies against the CaSR in the parathyroid glands were found in many patients with this abnormality. Adrenal insufficiency is a late phenomenon in this syndrome. Hyperparathyroidism is a recognized complication of thalassemia occurring after multiple transfusions and also has been described in patients with Wilson disease. Deposition of iron and copper in the parathyroid glands is the likely mechanism of parathyroid hypofunction in these patients (88). Also, parathyroid granulomas and metastatic cancer can lead to hypoparathyroidism.
Hypocalcemia may occur with magnesium depletion. Hypomagnesemia has been reported to induce skeletal resistance to PTH. It has been proposed that low serum magnesium diminishes the synthesis of PTH. It is interesting that some patients with hyperparathyroidism who exhibit resistance to vitamin D respond after administration of magnesium. Hypocalcemia associated with magnesium depletion responds poorly to intravenous calcium. Profound hypocalcemia may appear after therapeutic use of magnesium sulfate (e.g., in preeclampsia of pregnancy) because of suppression of PTH secretion. Certain drugs such as aminoglycosides and cytotoxic agents may have a direct toxic effect on parathyroid glands, leading to hypocalcemia. Irradiation of neck or administration of radioactive iodine also may affect parathyroid function (88). Symptomatic hyperparathyroidism also has been described in association with HIV infection.
IDIOPATHIC HYPOPARATHYROIDISM
Idiopathic hypoparathyroidism may be sporadic or familial. The familial congenital type is associated with hypocalcemic seizures in infancy. Familial idiopathic hypoparathyroidism is a heterogenous group of disorders. It may result from a mutation of the prepro-PTH gene or mutations of currently unidentified loci that affect the development, structure, or function of parathyroid glands (93, 94).
The human PTH is encoded by a single gene that was mapped to the short arm of chromosome 11. Mutations in this gene may lead to familial hypoparathyroidism with autosomal dominant transmission. The levels of PTH in serum may be low or undetectable in affected patients (93, 94).
The DiGeorge or velocardiaofacial syndrome consists of a congenital failure of development of derivatives of the third and fourth pharyngeal pouches, leading to absence of parathyroid glands and thymus. This syndrome may be inherited as an autosomal dominant disorder. It is associated with deletion of the long arm of chromosome 22 (95).
X-linked recessive hypoparathyroidism, like the DiGeorge syndrome, is associated with parathyroid agenesis and undetectable PTH levels in circulation. The X-linked recessive hypoparathyroidism gene was mapped to the distal long arm of the X chromosome (93, 94). Hypoparathyroidism may be caused by mutations or deletions in transcription factors or regulators of the development of parathyroid glands. Familial hypoparathyroidism due to dysgenesis of parathyroid glands results from mutations in the transcription factors glial cell missing B (GCMB) and glial cell missing 2 (GCM2). GCM2 is the master regulatory gene for parathyroid gland function and transcription factor for parathyroid gland development. A recent study has shown that one of the functions of GCM2 is to maintain high levels of CaSR expression in the parathyroid glands (96). Mutation in another transcription factor, globin transcription factor (GATA) protein-binding protein 3 (GATA3), which is a critical transcription factor for many developmental processes, causes an autosomal dominant complex disorder that combines hypoparathyroidism, deafness, and renal dysplasia.
A mutation in the gene coding for tubulin-specific chaperone E (TBCE), a peripheral membrane-associated tubulin-folding cofactor protein that is required for microtubule assembly, causes a rare autosomal recessive complex syndrome that includes hypoparathyroidism. The syndrome consists of hypoparathyroidism, retardation, and dysmorphism (HRD or Sanjad-Sakati syndrome). HRD is characterized by congenital hypoparathyroidism, intrauterine growth retardation, osteosclerosis, calcification of basal ganglia, mental retardation, seizures, and a typical facial dysmorphism featuring prominent forehead, deep-set eyes, and abnormal external ears. Mutation of the same gene was also reported in patients with autosomal recessive Kenny-Caffey syndrome (KCS). KCS resembles the HRD phenotype but is characterized by the presence of normal intelligence, late closure of the anterior fontanel, macrocephaly, postnatal growth retardation, and corneal opacity (97). Defects in maternal mitochondrial genes cause the Kearns-Sayre syndrome consisting of hypoparathyroidism, ophthalmoplegia, retinitis pigmentosa, cardiomyopathy with heart block, and diabetes mellitus.
Recently, autosomal dominant hypoparathyroidism was reported in families with activating mutations of the gene that encodes the extracellular CaSR in chromosome 3. In one family, missense mutation was found in the CaSR gene (98). These mutations cause excessive calcium-induced inhibition of PTH secretion. The hypocalcemia is mild and asymptomatic. It should be treated cautiously when mild because raising serum calcium concentrations markedly enhances urinary calcium excretions, with increasing risk of nephrocalcinosis and renal insufficiency.
PSEUDOHYPOPARATHYROIDISM
Pseudohypoparathyroidism is a rare inheritable disorder characterized by mental retardation, moderate obesity, short stature, brachydactyly with short metacarpal and metatarsal bones, exostoses, radius curves, and expressionless face. The biochemical abnormalities are hypocalcemia and hyperphosphatemia (99, 100). Some patients exhibit only the biochemical abnormalities. Thus, the disorder may be subdivided into pseudohypoparathyroidism type IA, which is also known as Albright hereditary osteodystrophy and IB.
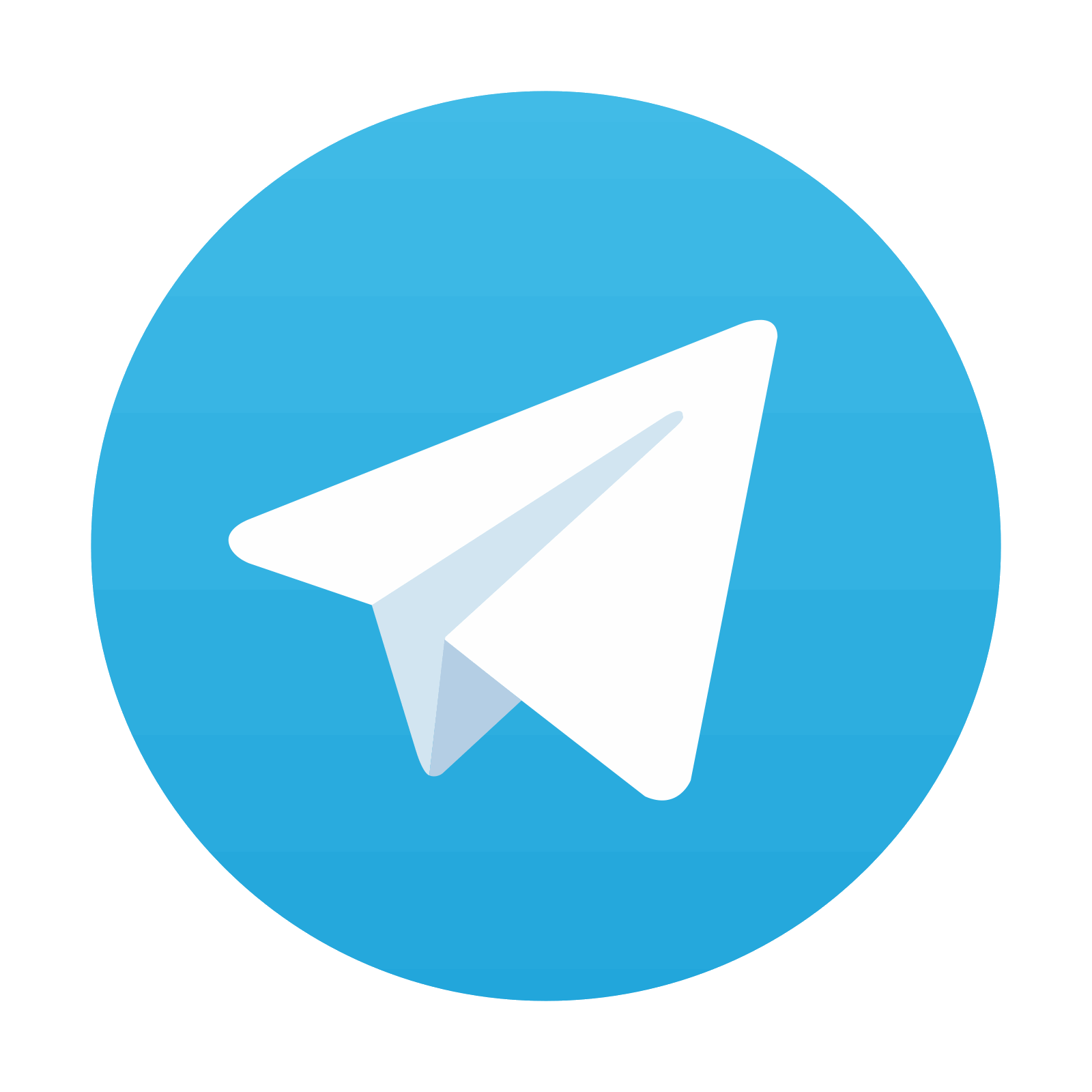
Stay updated, free articles. Join our Telegram channel
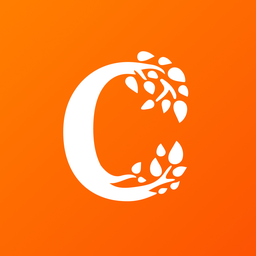
Full access? Get Clinical Tree
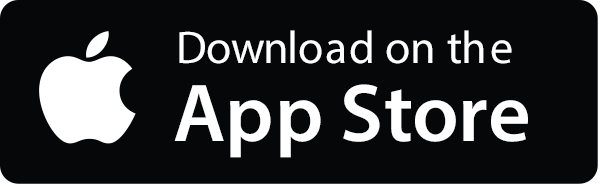
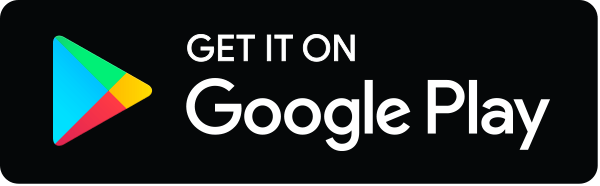