OBJECTIVES
After studying this chapter, you should be able to:
Define the special features of the circulation in the brain, coronary vessels, skin, and fetus, and how these are regulated.
Describe how cerebrospinal fluid (CSF) is formed and reabsorbed, and its role in protecting the brain from injury.
Understand how the blood-brain barrier impedes the entry of specific substances into the brain.
Delineate how the oxygen needs of the contracting myocardium are met by the coronary arteries, and the consequences of their occlusion.
List the vascular reactions of the skin and the reflexes that mediate them.
Understand how the fetus is supplied with oxygen and nutrients in utero, and the circulatory events required for a transition to independent life after birth.
INTRODUCTION
The distribution of the cardiac output to various parts of the body at rest in a normal man is shown in Table 33–1. The general principles described in preceding chapters apply to the circulation of all these regions, but the vascular supplies of many organs have additional special features that are important to their physiology. The portal circulation of the anterior pituitary is discussed in Chapter 18; the pulmonary circulation in Chapter 34; the renal circulation in Chapter 37; and the circulation of the splanchnic area, particularly the intestines and liver, in Chapters 25 and 28. This chapter is concerned with the special circulations of the brain, the heart, and the skin, as well as the placenta and fetus.
Blood Flow | Oxygen Consumption | Resistance (R units)a | Percentage of Total | |||||||
---|---|---|---|---|---|---|---|---|---|---|
Region | Mass (kg) | mL/min | mL/100 g/min | Arteriovenous Oxygen Difference (mL/L) | mL/min | mL/100 g/min | Absolute | per kg | Cardiac Output | Oxygen Consumption |
Liver | 2.6 | 1500 | 57.7 | 34 | 51 | 2.0 | 3.6 | 9.4 | 27.8 | 20.4 |
Kidneys | 0.3 | 1260 | 420.0 | 14 | 18 | 6.0 | 4.3 | 1.3 | 23.3 | 7.2 |
Brain | 1.4 | 750 | 54.0 | 62 | 46 | 3.3 | 7.2 | 10.1 | 13.9 | 18.4 |
Skin | 3.6 | 462 | 12.8 | 25 | 12 | 0.3 | 11.7 | 42.1 | 8.6 | 4.8 |
Skeletal muscle | 31.0 | 840 | 2.7 | 60 | 50 | 0.2 | 6.4 | 198.4 | 15.6 | 20.0 |
Heart muscle | 0.3 | 250 | 84.0 | 114 | 29 | 9.7 | 21.4 | 6.4 | 4.7 | 11.6 |
Rest of body | 23.8 | 336 | 1.4 | 129 | 44 | 0.2 | 16.1 | 383.2 | 6.2 | 17.6 |
Whole body | 63.0 | 5400 | 8.6 | 46 | 250 | 0.4 | 1.0 | 63.0 | 100.0 | 100.0 |
CEREBRAL CIRCULATION: ANATOMIC CONSIDERATIONS
The principal arterial inflow to the brain in humans is via four arteries: two internal carotid arteries and two vertebral arteries. In humans, the carotid arteries are quantitatively the most significant. The vertebral arteries unite to form the basilar artery, and the basilar artery and the carotids form the circle of Willis below the hypothalamus. The circle of Willis is the origin of the six large vessels supplying the cerebral cortex. Substances injected into one carotid artery are distributed almost exclusively to the cerebral hemisphere on that side. Normally no crossing over occurs, probably because the pressure is equal on both sides. Even when it is not, the anastomotic channels in the circle do not permit a very large flow. Occlusion of one carotid artery, particularly in older patients, often causes serious symptoms of cerebral ischemia. There are precapillary anastomoses between the cerebral vessels, but flow through these channels is generally insufficient to maintain the circulation and prevent infarction when a cerebral artery is occluded.
Venous drainage from the brain by way of the deep veins and dural sinuses empties principally into the internal jugular veins in humans, although a small amount of venous blood drains through the ophthalmic and pterygoid venous plexuses, through emissary veins to the scalp, and down the system of paravertebral veins in the spinal canal.
The cerebral vessels have a number of unique anatomic features. In the choroid plexuses, there are gaps between the endothelial cells of the capillary wall, but the choroid epithelial cells that separate them from the cerebrospinal fluid (CSF) are connected to one another by tight junctions. The capillaries in the brain substance resemble nonfenestrated capillaries in muscle (see Chapter 31), but there are tight junctions between the endothelial cells that limit the passage of substances via the paracellular route. In addition, there are relatively few vesicles in the endothelial cytoplasm, and presumably little vesicular transport. However, multiple transport systems are present in the capillary cells. The brain capillaries are surrounded by the endfeet of astrocytes (Figure 33–1). These endfeet are closely applied to the basal lamina of the capillaries, but they do not cover the entire capillary wall, and gaps of about 20 nm occur between endfeet (Figure 33–2). However, the endfeet induce the tight junctions in the capillaries (see Chapter 31). The protoplasm of astrocytes is also found around synapses, where it appears to isolate the synapses in the brain from one another.
FIGURE 33–1
Relation of fibrous astrocyte (3) to a capillary (2) and neuron (4) in the brain. The endfeet of the astrocyte processes form a discontinuous membrane around the capillary (1). Astrocyte processes also envelop the neuron. (Adapted with permission from Krstic RV: Die Gewebe des Menschen und der Säugetiere. Springer, 1978.)
Three systems of nerves innervate the cerebral blood vessels. Postganglionic sympathetic neurons have their cell bodies in the superior cervical ganglia, and their endings contain norepinephrine. Many also contain neuropeptide Y. Cholinergic neurons that probably originate in the sphenopalatine ganglia also innervate the cerebral vessels, and the postganglionic cholinergic neurons on the blood vessels contain acetylcholine. Many also contain vasoactive intestinal peptide (VIP) and peptide histidyl methionine (PHM-27) (see Chapter 7). These nerves end primarily on large arteries. Sensory nerves are found on more distal arteries. They have their cell bodies in the trigeminal ganglia and contain substance P, neurokinin A, and calcitonin gene-related peptide (CGRP). Substance P, CGRP, VIP, and PHM-27 cause vasodilation, whereas neuropeptide Y is a vasoconstrictor. Touching or pulling on the cerebral vessels causes pain.
CEREBROSPINAL FLUID
CSF fills the ventricles and subarachnoid space. In humans, the volume of CSF is about 150 mL and the rate of CSF production is about 550 mL/d. Thus the CSF turns over about 3.7 times a day. In experiments on animals, it has been estimated that 50–70% of the CSF is formed in the choroid plexuses and the remainder is formed around blood vessels and along ventricular walls. Presumably, the situation in humans is similar. The CSF in the ventricles flows through the foramens of Magendie and Luschka to the subarachnoid space and is absorbed through the arachnoid villi into veins, primarily the cerebral venous sinuses. The villi consist of projections of the fused arachnoid membrane and endothelium of the sinuses into the venous sinuses. Similar, smaller villi project into veins around spinal nerve routes. These projections may contribute to the outflow of CSF into venous blood by a process known as bulk flow, which is unidirectional. However, recent studies suggest that, at least in animals, a more important route for CSF reabsorption into the bloodstream in health is via the cribriform plate above the nose and thence into the cervical lymphatics. However, reabsorption via one-way valves (of uncertain structural basis) in the arachnoid villi may assume a greater role if CSF pressure is elevated. Likewise, when CSF builds up abnormally, aquaporin water channels may be expressed in the choroid plexus and brain microvessels as a compensatory adaptation.
CSF is formed continuously by the choroid plexus in two stages. First, plasma is passively filtered across the choroidal capillary endothelium. Next, secretion of water and ions across the choroidal epithelium provides for active control of CSF composition and quantity. Bicarbonate, chloride, and potassium ions enter the CSF via channels in the epithelial cell apical membranes. Aquaporins provide for water movement to balance osmotic gradients. The composition of CSF (Table 33–2) is essentially the same as that of brain extracellular fluid (ECF), which in living humans makes up 15% of the brain volume. In adults, free communication appears to take place between the brain interstitial fluid and CSF, although the diffusion distances from some parts of the brain to the CSF are appreciable. Consequently, equilibration may take some time to occur, and local areas of the brain may have extracellular microenvironments that are transiently different from CSF.
Units | CSF | Plasma | Ratio CSF/Plasma | |
---|---|---|---|---|
Na+ | (mEq/kg H2O) | 147.0 | 150.0 | 0.98 |
K+ | (mEq/kg H2O) | 2.9 | 4.6 | 0.62 |
Mg2+ | (mEq/kg H2O) | 2.2 | 1.6 | 1.39 |
Ca2+ | (mEq/kg H2O) | 2.3 | 4.7 | 0.49 |
Cl– | (mEq/kg H2O) | 113.0 | 99.0 | 1.14 |
HCO3– | (mEq/L) | 25.1 | 24.8 | 1.01 |
Pco2 | (mm Hg) | 50.2 | 39.5 | 1.28 |
pH | 7.33 | 7.40 | … | |
Osmolality | (mOsm/kg H2O) | 289.0 | 289.0 | 1.00 |
Protein | (mg/dL) | 20.0 | 6000.0 | 0.003 |
Glucose | (mg/dL) | 64.0 | 100.0 | 0.64 |
Inorganic P | (mg/dL) | 3.4 | 4.7 | 0.73 |
Urea | (mg/dL) | 12.0 | 15.0 | 0.80 |
Creatinine | (mg/dL) | 1.5 | 1.2 | 1.25 |
Uric acid | (mg/dL) | 1.5 | 5.0 | 0.30 |
Cholesterol | (mg/dL) | 0.2 | 175.0 | 0.001 |
Lumbar CSF pressure is normally 70–180 mm H2O. Up to pressures well above this range, the rate of CSF formation is independent of intraventricular pressure. However, absorption is proportional to the pressure (Figure 33–3). At a pressure of 112 mm H2O, which is the average normal CSF pressure, filtration and absorption are equal. Below a pressure of approximately 68 mm H2O, absorption stops. Large amounts of fluid accumulate when the capacity for CSF reabsorption is decreased (external hydrocephalus, communicating hydrocephalus). Fluid also accumulates proximal to the block and distends the ventricles when the foramens of Luschka and Magendie are blocked or there is obstruction within the ventricular system (internal hydrocephalus, noncommunicating hydrocephalus).
FIGURE 33–3
CSF formation and absorption in humans at various CSF pressures. Note that at 112 mm CSF, formation and absorption are equal, and at 68 mm CSF, absorption is zero. CSF, cerebrospinal fluid. (Modified with permission from Cutler RWP, et al: Formation and absorption of cerebrospinal fluid in man. Brain 1968; 91(4):707–720.)
The most critical role for CSF (and the meninges) is to protect the brain. The dura is attached firmly to bone. Normally, there is no “subdural space,” with the arachnoid being held to the dura by the surface tension of the thin layer of fluid between the two membranes. As shown in Figure 33–4, the brain itself is supported within the arachnoid by the blood vessels and nerve roots and by the multiple fine fibrous arachnoid trabeculae. The brain weighs about 1400 g in air, but in its “water bath” of CSF it has a net weight of only 50 g. The buoyancy of the brain in the CSF permits its relatively flimsy attachments to suspend it very effectively. When the head receives a blow, the arachnoid slides on the dura and the brain moves, but its motion is gently checked by the CSF cushion and by the arachnoid trabeculae.
The pain produced by spinal fluid deficiency illustrates the importance of CSF in supporting the brain. Removal of CSF during lumbar puncture can cause a severe headache after the fluid is removed, because the brain hangs on the vessels and nerve roots, and traction on them stimulates pain fibers. The pain can be relieved by intrathecal injection of sterile isotonic saline.
Without the protection of the spinal fluid and the meninges, the brain would probably be unable to withstand even the minor traumas of everyday living; but with the protection afforded, it takes a fairly severe blow to produce cerebral damage. The brain is damaged most commonly when the skull is fractured and bone is driven into neural tissue (depressed skull fracture), when the brain moves far enough to tear the delicate bridging veins from the cortex to the bone, or when the brain is accelerated by a blow on the head and is driven against the skull or the tentorium at a point opposite where the blow was struck (contrecoup injury).
THE BLOOD-BRAIN BARRIER
The tight junctions between capillary endothelial cells in the brain and between the epithelial cells in the choroid plexus effectively prevent proteins from entering the brain in adults and slow the penetration of some smaller molecules as well. An example is the slow penetration of urea (Figure 33–5). This uniquely limited exchange of substances into the brain is referred to as the blood-brain barrier, a term most commonly used to encompass this barrier overall and more specifically the barrier in the choroid epithelium between blood and CSF.
Passive diffusion across the tight cerebral capillaries is very limited, and little vesicular transport takes place. However, there are numerous carrier-mediated and active transport systems in the cerebral capillaries. These move substances out of as well as into the brain, though movement out of the brain is generally freer than movement into it.
Water, CO2, and O2 penetrate the brain with ease, as do the lipid-soluble free forms of steroid hormones, whereas their protein-bound forms and, in general, all proteins and polypeptides do not. The rapid passive penetration of CO2 contrasts with the regulated transcellular penetration of H+ and HCO3– and has physiologic significance in the regulation of respiration (see Chapter 35).
Glucose is the major ultimate source of energy for nerve cells. Its diffusion across the blood-brain barrier would be very slow, but the rate of transport into the CSF is markedly enhanced by the presence of specific transporters, including the glucose transporter 1 (GLUT1). The brain contains two forms of GLUT1: GLUT1 55K and GLUT1 45K. Both are encoded by the same gene, but they differ in the extent to which they are glycosylated. GLUT1 55K is present in high concentration in brain capillaries (Figure 33–6). Infants with congenital GLUT1 deficiency have low CSF glucose concentrations in the presence of normal plasma glucose, and they have seizures and delayed development. In addition, transporters for thyroid hormones; several organic acids; choline; nucleic acid precursors; and neutral, basic, and acidic amino acids are present at the blood-brain barrier.
A variety of drugs and peptides actually cross the cerebral capillaries but are promptly transported back into the blood by a multidrug nonspecific transporter in the apical membranes of the endothelial cells. This P-glycoprotein is a member of the family of adenosine triphosphate (ATP) binding cassettes that transport various proteins and lipids across cell membranes (see Chapter 2). In the absence of this transporter in mice, much larger proportions of systemically administered doses of various chemotherapeutic drugs, analgesics, and opioid peptides are found in the brain than in controls. If pharmacologic agents that inhibit this transporter can be developed, they could be of value in the treatment of brain tumors and other central nervous system (CNS) diseases in which it is difficult to introduce adequate amounts of therapeutic agents into the brain.
When dyes that bind to proteins in the plasma are injected, they stain many tissues but spare most of the brain. However, four small areas in or near the brainstem do take up the stain. These areas are (1) the posterior pituitary (neurohypophysis) and the adjacent ventral part of the median eminence of the hypothalamus, (2) the area postrema, (3) the organum vasculosum of the lamina terminalis (OVLT, supraoptic crest), and (4) the subfornical organ (SFO).
These areas are referred to collectively as the circumventricular organs (Figure 33–7). All have fenestrated capillaries, and because of their permeability they are said to be “outside the blood-brain barrier.” Some of them function as neurohemal organs; that is, areas in which polypeptides secreted by neurons enter the circulation. Others contain receptors for many different peptides and other substances, and function as chemoreceptor zones in which substances in the circulating blood can act to trigger changes in brain function without penetrating the blood-brain barrier. For example, the area postrema is a chemoreceptor trigger zone that initiates vomiting in response to chemical changes in the plasma (see Chapter 27). It is also concerned with cardiovascular control, and in many species circulating angiotensin II acts on the area postrema to produce a neurally mediated increase in blood pressure. Angiotensin II also acts on the SFO and possibly on the OVLT to increase water intake. In addition, it appears that the OVLT is the site of the osmoreceptor controlling vasopressin secretion (see Chapter 38), and evidence suggests that circulating interleukin-1 (IL-1) produces fever by acting here too.
FIGURE 33–7
Circumventricular organs. The neurohypophysis (NH), organum vasculosum of the lamina terminalis (OVLT, organum vasculosum of the lamina terminalis), subfornical organ (SFO), and area postrema (AP) are shown projected on a sagittal section of the human brain. PI, pineal; SCO, subcommissural organ.
The subcommissural organ (Figure 33–7) is closely associated with the pineal gland and histologically resembles the circumventricular organs. However, it does not have fenestrated capillaries, is not highly permeable, and has no established function. Conversely, the pineal and the anterior pituitary do have fenestrated capillaries and are outside the blood-brain barrier, but both are endocrine glands and are not part of the brain.
The blood-brain barrier strives to maintain the constancy of the environment of the neurons in the CNS (Clinical Box 33–1). Even minor variations in the concentrations of K+, Ca2+, Mg2+, H+, and other ions can have far-reaching consequences. The constancy of the composition of the ECF in all parts of the body is maintained by multiple homeostatic mechanisms (see Chapters 1 and 38), but because of the sensitivity of the cortical neurons to ionic change, it is not surprising that an additional defense has evolved to protect them. Other functions of the blood-brain barrier include protection of the brain from endogenous and exogenous toxins in the blood and prevention of the escape of neurotransmitters into the general circulation.
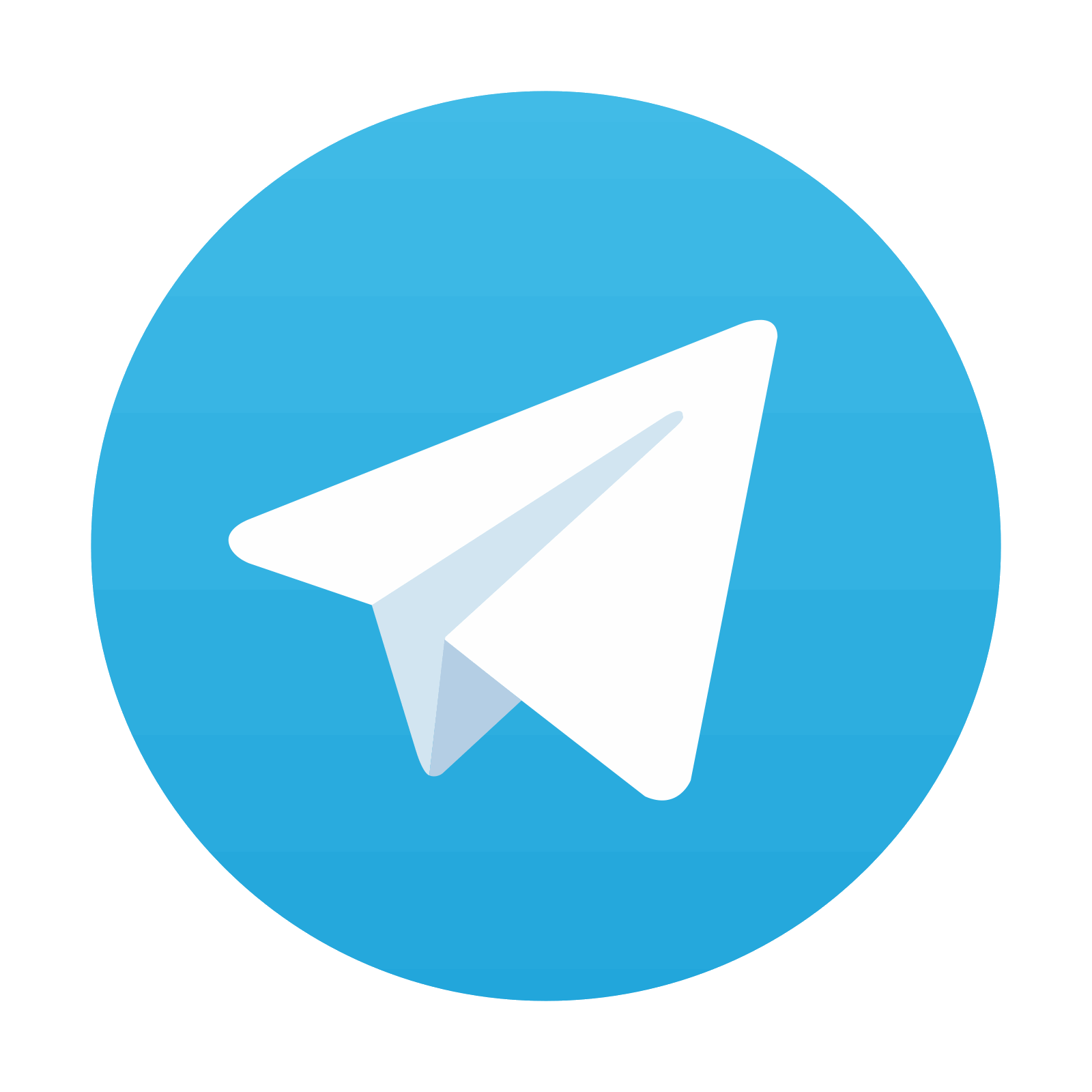
Stay updated, free articles. Join our Telegram channel
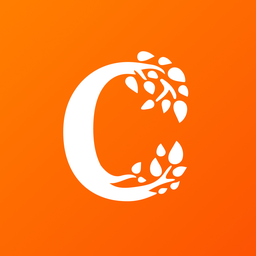
Full access? Get Clinical Tree
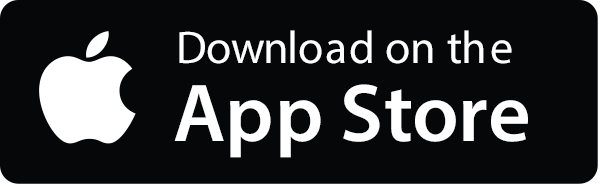
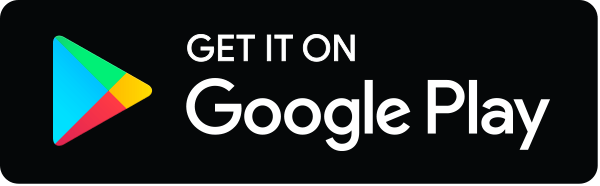