Overview
The respiratory system facilitates the exchange of oxygen and carbon dioxide between the external environment and the human body. The airways (trachea and bronchi), lungs, and alveoli and pulmonary surfactant provide the biochemical and biophysical basis for this exchange. Acid–base balance in the body also relies on these tissues as well as on the carbonic anhydrase and other enzyme systems. Various diseases of the respiratory system affect either the structure or the biochemistry of the lungs; medications and treatments for these diseases rely on knowing their biochemical basis.
Basic Anatomy and Development
The respiratory system, composed of the nose, nasal cavity, larynx, trachea, bronchiole tubes, and lungs, is derived from the endoderm, although the splanchnic mesoderm contributes to the visceral pleura, cartilage, and connective tissue and also to the immediately associated smooth musculature and capillaries (Figure 17-1). Other closely associated structures include the ribs and diaphragm and the central cardiovascular system, which provides pulmonary arteries and veins.
Figure 17-1.
Overview of Lung Structure. The basic structure of lungs (A) includes the trachea and repetitively branching bronchi/bronchioles leading to a terminal bronchiole. Pulmonary arteries and veins provide circulation to this lung tissue. At the terminal bronchiole/alveoli (B), the exchange of oxygen and carbon dioxide between alveoli and the surrounding vasculature is conducted as discussed later in this chapter. [Reproduced with permission from Barrett KE, et al.: Ganong’s Review of Medical Physiology, 23rd edition, McGraw-Hill, 2010.]
The respiratory system facilitates the transport of oxygen (O2) to the body’s tissues and enables elimination of gaseous waste products of metabolism. Muscles of the ribs, diaphragm, neck, shoulders, and abdomen generate a negative pressure gradient, which promotes the flow of external air into a system of branching, conducting airways and peripheral terminal bronchioles.
Exchange via passive diffusion through the cell membranes of the type I epithelial cells of the alveoli and the pulmonary capillaries is responsible for the uptake and removal of O2 and carbon dioxide (CO2), respectively (Figure 17-2). Pulmonary arteries from the right ventricle of the heart deliver deoxygenated, CO2-laden blood through the branching blood vessels, to the capillaries; pulmonary veins return the freshly oxygenated blood with decreased CO2 to the left auricle and ventricle for delivery to the body’s tissues. Finally, positive pressure, along with the elastic walls of the alveoli, springing back from full expansion, expels the waste gas, including the CO2. Although exhalation is normally a passive process, active expulsion is also possible, utilizing the same muscle groups noted above. Defects or injuries to any of the parts of the respiratory system or to those that affect the musculature and/or cardiovascular system can lead to respiratory disease.
Figure 17-2.
Structure of the Alveolus. (A) The alveolus allows the exchange of oxygen (O2) and carbon dioxide (CO2) between the alveolar air and capillary. (B) Magnified view of the structure of the alveolar/pulmonary capillary interface showing the proximity of alveolar air and the capillaries. This structure allows the efficient diffusion of O2 and CO2 through the single-cell layer of type I cells to/from erythrocytes in the capillaries and the alveolus. The pulmonary interstitium and type II cells responsible for secretion of surfactant, which helps to maintain the fragile structure of the alveoli, are also shown. [Adapted with permission from Kibble JD and Halsey CR: The Big Picture: Medical Physiology, 1st edition, McGraw-Hill, 2009.]
The passive diffusion of O2 and CO2 relies on the close proximity, cellular membranes, and interwoven connective tissues (collagen and elastin fibers; see Chapter 13) that allow the alveolar–capillary gas exchange. The overall large surface area of the approximately 300 million alveoli in the average human body provides roughly 75 m2 of gas exchange area. The capillary network that surrounds these alveoli covers about 70% of this area. Gas exchange between the alveoli and the pulmonary capillary is driven by the higher “partial pressure” in the alveoli versus in the pulmonary capillaries. This pressure gradient propels the small O2 and CO2 through the basement membrane and the lipid bilayer of the alveolar type I squamous epithelial cell (∼0.2 μm thin) and the capillaries one layer of endothelial cell plasma membranes.
Pulmonary surfactant, composed of lecithin and myelin, is secreted on a continuous basis by type II alveolar cells and Clara cells beginning at approximately week 20 of gestation. Pulmonary surfactant has both a lipid (∼90% of total) and a protein (∼10% of total) component. About half of the lipids are dipalmitoylphosphatidylcholine (Chapter 4), the 16-carbon saturated lipid with a charged amine group on its head group. The remaining lipids include phosphatidylglycerol, which modulates the fluidity of the surfactant as well as cholesterol and other lipids. About half of the proteins are apoproteins, which have both hydrophobic and hydrophilic portions; the other half is composed of proteins normally found in blood plasma. These lipids and proteins all have the capability of interacting with aqueous (hydrophilic) or nonaqueous (hydrophobic) environments and it is this quality that leads to their specialized function in the lung (Figure 17-2). By directly interacting with alveolar water via their hydrophilic regions while the hydrophobic regions remain in the air, pulmonary surfactant creates a myelin meshwork that lines the alveoli—a strong, intertwined lipoprotein system that is analogous to the myelin sheath of nerve cells (Chapter 19).
This unique alveolar lining greatly reduces surface tension, allowing easier expansion/stretching (known as pulmonary compliance) and collapse of alveoli during respiration and the resulting changes in pressure. This reduction in surface tension decreases the work of respiration and the total amount of pressure that must be generated for efficient and effective inspiration and expiration. The pulmonary surfactant also helps all the lung alveoli to expand (inspiration) and shrink (expiration) at the same rate, thereby reducing the chance for isolated overexpansion and the total collapse (atelectasis) of the alveolar sacs.
Pulmonary Surfactant and Premature Birth: The respiratory system develops only partially during pregnancy and becomes fully functional only after birth. One medical consequence is seen in premature infants in whom pulmonary surfactant has only started to form. Specifically, premature babies born before 32 weeks of gestation must be treated aggressively before birth to help augment surfactant or it may result in Infant Respiratory Distress Syndrome (IRDS). This condition, also known as Hyaline Membrane Disease because of its findings on pathological examination, results in respiratory distress of the newborn infant along with accessory muscle use, grunting, nostril flaring, and cyanosis and, if not appropriately treated, cessation of breathing and death. Multiple complications can also result from IRDS, making it the leading cause of death of infants up to 1 month old in the developed world. The most successful treatment is frequent steroid injections to the mother, which prompts surfactant production while delaying birth for as long as possible. |
O2–CO2 Exchange in the Lung and Acid–Base Balance
A major function of blood and red blood cells is the transport of O2 to cells and CO2 from cells from/to the lung, respectively. As a result, diseases involving this system will often result in poor oxygenation (hypoxia) and/or increased CO2 levels (hypercapnia) of the blood and tissues. Approximately 60% of CO2 is carried back to the lungs dissolved in the blood plasma as bicarbonate molecules (HCO3−). The binding of O2 and exchange with CO2 is discussed in detail in Chapter 14.
The impact of O2–CO2 exchange extends beyond simple oxygenation of tissues and elimination of waste CO2. Levels of CO2, as regulated by the lung, kidneys (Chapter 18), blood (Chapter 14), and even the pancreas (Chapter 11), are responsible for maintaining proper and acceptable levels of hydrogen ions (H+) and, therefore, the body’s acid–base balance (Figure 17-3). Ranges of pH outside the normal body with a range of less than 7.35 (acidosis) or more than 7.45 (alkalosis), if uncompensated, can quickly lead to death. This balance is critical in the central nervous system where tissues and cells are even more sensitive to pH variations.
Figure 17-3.
Regulation of Acid–Base Balance by the Lung. Elimination of excess carbon dioxide (CO2) can occur by increased respiratory rate (hyperpnea), which removes bicarbonate (HCO3−) and hydrogen ions (H+) from other tissues (e.g., red blood cells). See the text for further details. [Reproduced with permission from Kibble JD and Halsey CR: The Big Picture: Medical Physiology, 1st edition, McGraw-Hill, 2009.]
Carbonic anhydrase (Figure 17-4), found in several tissues including the lungs, kidneys, gastrointestinal tract, saliva, brain, and red blood cells, is essential for the rapid conversion of CO2 to HCO3− (then carried in the plasma) and for the slower reverse reaction of HCO3− to H2CO3 (carbonic acid) to CO2 (Figure 17-3) in the lungs or kidneys. About 75% of the carbon in the body is in the form of H2CO3 or HCO3−. In the absence of carbonic anhydrase, an equilibrium of the components of the CO2 to HCO3− interconversion (Figure 17-4) is established. Carbonic anhydrase allows the body to bypass the carbonic acid step. In the lungs, carbonic anhydrase also facilitates HCO3− to CO2 via H2CO3.
Figure 17-4.
Interconversion of Carbon Dioxide (CO2) to Bicarbonate. CO2 is reversibly converted to bicarbonate ion (HCO3−) via the action of the enzyme carbonic anhydrase. Carbonic acid (H2CO3) is a bypassed intermediate in both reaction directions. Water molecule (H2O), essential to the process, and hydrogen ions (H+) are also shown.
Deficiencies in the management of CO2/bicarbonate in the lungs can lead to the medical conditions of respiratory acidosis and respiratory alkalosis. Both have acute and chronic forms. Acute respiratory acidosis occurs when there is a short-term decrease in respiratory function (hypoventilation), leading to a decrease in the amount of CO2 exhaled, and because CO2 is always being produced by the body, an increase in HCO3− per the reaction below (Figure 17-4). Chronic respiratory problems such as those caused by chronic obstructive pulmonary disease (COPD), interstitial lung diseases (see below), mechanical abnormalities (e.g., diaphragm dysfunction, deformities of the chest), respiratory muscle fatigue (e.g., chronic lung disease, extended asthma attack), or other disorders with longer term hypoventilation, increase CO2 in the blood, and result in higher concentrations of H+ (acidosis). Alternatively, acute or chronic increases in exhalation of CO2 with lowering of H+ concentrations (alkalosis) can result from lung (pneumonia and fever with increased rate of breathing), psychiatric (stress and anxiety), and/or neurological (stroke, meningitis) diseases, pregnancy, liver disease, overuse of aspirin and/or caffeine, recent increases in altitude, and even overly aggressive mechanical ventilation of patients. Various mechanisms attempt to compensate for any respiratory acidosis or alkalosis. Cells are able to either take up or release bicarbonate as part of a buffering system that can respond on a time scale of minutes. Red blood cells have a specific membrane transport protein for the movement of bicarbonate ions (Chapter 14). The kidneys are also able to increase or decrease bicarbonate excretion or reabsorption in the proximal tubules over a period of a few days (Chapter 18). Acid–base balance is discussed in more detail in Chapters 14 and 18.
Noninfective Diseases of the Respiratory System
Noninfective diseases of the respiratory system are grouped into obstructive and restrictive, although patients can suffer from one or both. Obstructive lung diseases
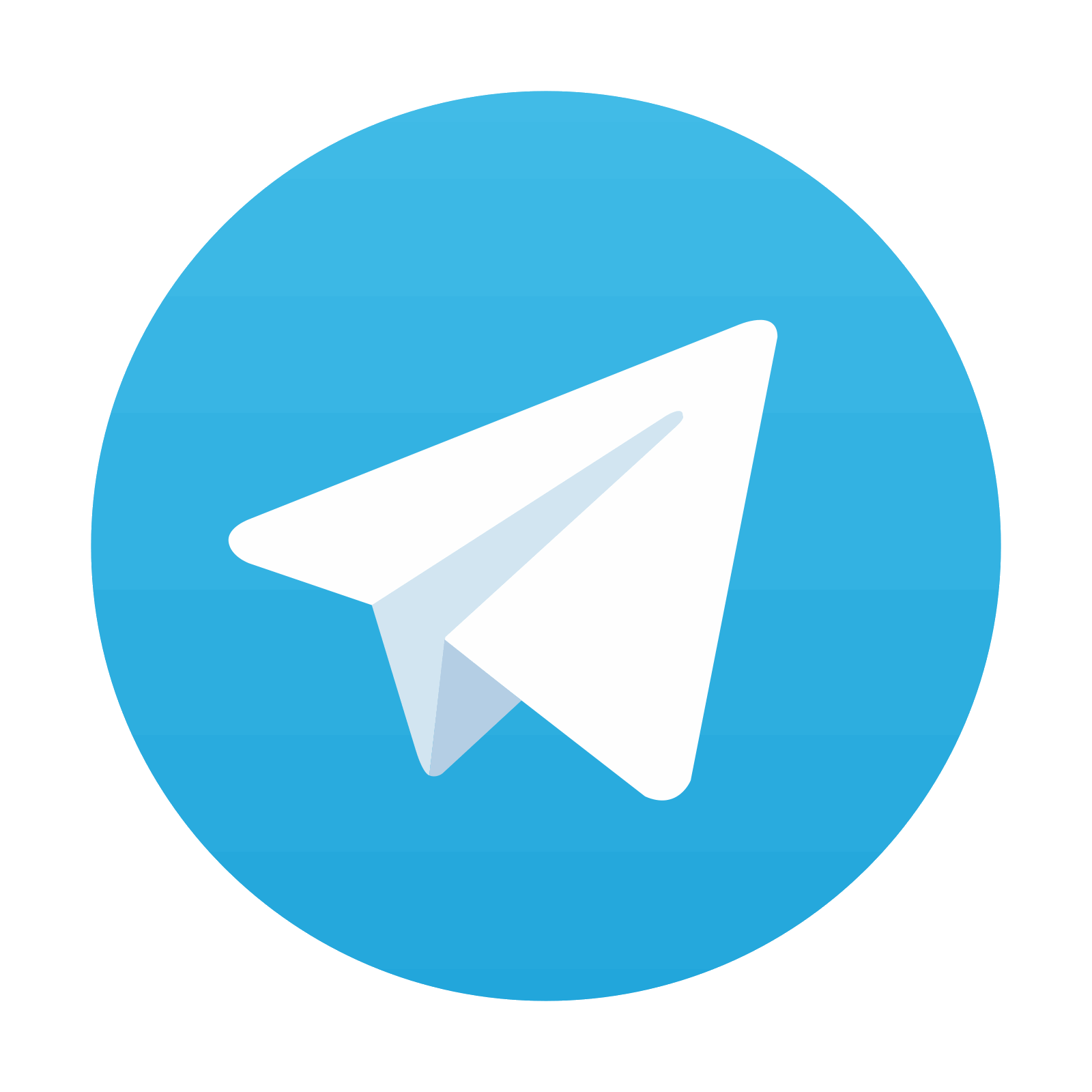
Stay updated, free articles. Join our Telegram channel
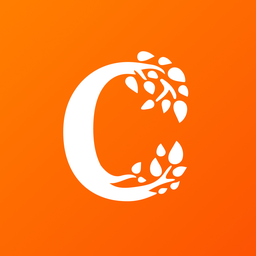
Full access? Get Clinical Tree
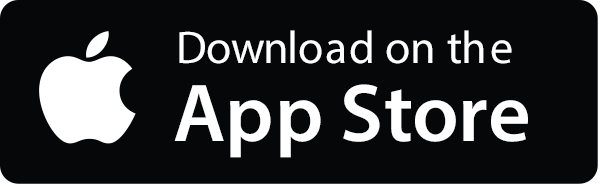
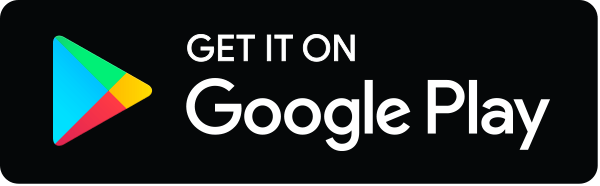
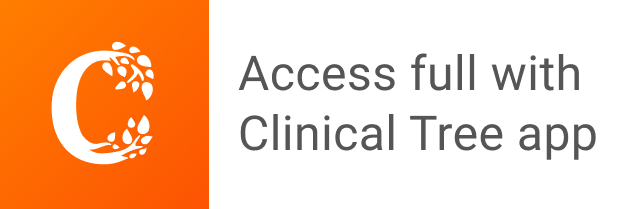