Biologic Basis of Gastric Colonization by Helicobacter
Introduction
The eradication of H. pylori for treatment of peptic ulcer disease not associated with NSAID use, steroids, or severe stress is now accepted as a necessary part of medical treatment of this set of illnesses. Perhaps less well accepted is the need for eradication of any infection by this gastric carcinogen. Since the early findings that infection was associated with fundic cell metaplasia and cancer, overwhelming data have been presented proving an association. For example, in Japan, where gastric carcinoma is the most common form of cancer, 3% of adult males older than 60 years who have infection have cancer, whereas none who are not infected has this lesion. A Swedish study showed a much higher probability of stomach cancer in patients undergoing hip transplant who were infected than in those who were uninfected. Even more germane, in a subgroup of patients who had been given high-dose antibiotics as coverage for this surgery, the incidence of cancer dropped precipitously, indicating that eradication is of benefit even after many years of infection.
Currently, therapy with PPIs and two antibiotics is the most frequently prescribed medication, with perhaps bismuth along with ranitidine and also two antibiotics as another possible therapy. The rationale for the need for the former combination is becoming clearer, but monotherapy would be more desirable, simplifying treatment and avoiding the use of antibiotics necessary for the treatment of other infections. To understand the biologic basis of this infection and pave the way for better treatment, the biology of H. pylori is being investigated on many fronts.
The nature of Helicobacter pylori
There has been much discussion about the pH of the environment inhabited by H. pylori. Although some would have the gastric surface neutral in spite of several hours with a luminal pH of approximately 1.0, most would agree that with a luminal pH of less than 2.0, surface pH is also 2.0 or less. Hence, H. pylori thrives in an acidic environment.
Bacteria can inhabit a variety of pH environments: acidic (acidophiles), alkaline (alkalophiles), and neutral (neutralophiles). In spite of the varying acidity of the environment, all bacteria harness the energy of the electrochemical proton gradient across their membranes to synthesize ATP. This gradient consists of a pH gradient and a gradient of electric potential difference. Hence, this gradient is modified by the different types of bacteria. Acidophiles already have an inward pH gradient under acidic conditions and modify their membrane potential so that it becomes internally positive to restrict inward proton movement. Alkalophiles increase their inside negative potential, so that the pH inward driving force is magnified. Neutralophiles grow best between pH 6.0 and 8.0 and do not grow outside this range.
The latter finding is also true of H. pylori in the absence of specific additions to the medium, such as urea. Neutralophiles are generally able to survive an
acidic environment but do not grow in acid. Depending on whether they are in growth or log phase or in stationary phase, the response is considered acid tolerant or acid resistant. In either case, this allows the organisms to maintain viability. H. pylori and other gastric Helicobacter species are unique in that they not only survive but also grow in the gastric environment. These are, therefore, the only bacteria that have adapted to an acidic environment.
acidic environment but do not grow in acid. Depending on whether they are in growth or log phase or in stationary phase, the response is considered acid tolerant or acid resistant. In either case, this allows the organisms to maintain viability. H. pylori and other gastric Helicobacter species are unique in that they not only survive but also grow in the gastric environment. These are, therefore, the only bacteria that have adapted to an acidic environment.
Aerobic bacteria (including microaerobes) synthesize ATP by coupling metabolic generation and oxidation of substrates to the formation of an electrochemical proton gradient across their cytoplasmic membrane (pH and membrane potential) and dissipation of this proton gradient through the cytoplasmic membrane’s ATP synthase. The proton gradient is composed of two components: an actual pH gradient and a potential difference across the membrane. A balance of these two constituents of the proton energy charge of the membrane enables bacterial growth over a range of pH 2.0 to 11.0, depending on whether they are acidophiles, neutralophiles, or alkalophiles.
The outer membrane contains porins, which usually allow passage of protons. An acid-dwelling organism can either accept high acidity in the periplasmic space and regulate H+ entry by changing the transmembrane potential or it can choose to regulate the pH of the periplasmic space, thus changing the pH component.
The circulation of protons across the cytoplasmic membrane is electrogenic, that is to say that export of protons by the redox pumps oxidizing substrate generates current and voltage cytoplasmic side negative. Uptake of protons through the F1F0 ATPase driven by the pH and electrical gradient dissipates the potential and the pH and uses this to generate ATP from ADP and Pi.
Formally, the gradient of hydrogen ions is expressed as a function of the chemical and electrical potential, in which the electrochemical gradient of hydrogen ions is the driving force for ATP generation by the chemiosmotic mechanism first recognized by Peter Mitchell in 1961. The thermodynamic equation describing the electrochemical gradient (proton motive force) for H+ is

where
is the electrochemical gradient for protons, R is the gas constant, T is the temperature in degrees Kelvin, F is the Faraday constant, n is the valence of the ion, and Δψ is the transmembrane potential, referred to in text as PD.

This relationship predicts that there is a reciprocal relationship between the pH gradient and the potential difference (i.e., as the inward pH gradient increases, the PD decreases, and vice versa, to maintain a relatively constant proton motive force). The chemiosmotic mechanism for ATP generation by a neutralophile is illustrated in the figure.
Because mean intragastric pH in people is 1.4, the issue arises as to the bioenergetic nature of H. pylori: is it an acidophile that has learned to colonize the human stomach? Or is it a neutralophile that has developed adaptive mechanisms to combat the variable acidity of its environment? Because it grows best between pH 6.0 and 8.0 and does not survive either pH less than 4.0 or pH greater than 8.2 in the absence of pH-regulatory enzymes such as urease, it is clearly a neutralophile but may have adopted some of the mechanisms of acidophiles to be able to inhabit the stomach.
H. pylori is a motile, gram-negative organism that can be cultured in microaerobic (low O2) conditions, although it adapts to high O2 at higher culture densities. As such, it has an outer membrane, the outer leaflet of which is lipopolysaccharide, a cell wall and periplasm, an inner membrane, and cytoplasm. The bioenergetic survival of the organism depends on the maintenance of an adequate proton motive force between the periplasmic space and the cytoplasm across its inner or cytoplasmic membrane.
The organism is helical or spiral in shape and possesses six to eight flagella at one end. Flagellar function depends on the activity of a flagellar motor also driven by the proton motive force generated across the cytoplasmic membrane of the organism. Given the two-membrane structure of the organism, control of periplasmic and cytoplasmic pH is vital for survival and growth of the organism.
Modern molecular biologic methods for defining the genome have been applied to the genetic structure of H. pylori. The genome contains approximately 1,500 genes, 300 of which encode membrane proteins, many of as-yet unknown function. The genome contains sequences encoding for membrane proteins, such as the F1F0 ATP synthase complex, and various oxidoreductases,
such as cytochrome o, several transporters, and a variety of two component signaling systems (the equivalent of eukaryotic receptors). Some of the recognized transporters are illustrated in the figure on the previous page.
such as cytochrome o, several transporters, and a variety of two component signaling systems (the equivalent of eukaryotic receptors). Some of the recognized transporters are illustrated in the figure on the previous page.
![]() Electron micrograph of H. pylori, showing outer and inner membranes separated by the periplasm and the polar location of the flagellae. |
The organism contains enzymes for glucose metabolism but lacks β-galactosidase (and hence is unable to metabolize lactose) and some of the enzymes of the Krebs cycle (no isocitrate dehydrogenase). The genome also encodes for several outer membrane proteins (OMPs), some of which are porins, able to allow movement of a variety of molecules into or out of the periplasmic space. Some of these have a higher isoelectric point than those of neutralophiles such as E. coli or B. subtilis, which may enable resistance to acute acidic changes of pH, because proton permeability is expected to be less because they are more positively charged.
Acid and Helicobacter pylori
The survival and growth of organisms as a function of medium pH is diagnostic of their bioenergetic profile. For example, neutralophiles such as E. coli characteristically are able to survive between pH 4.0 and 8.0 and grow well between pH 6.0 and 8.0. When these properties of H. pylori are measured as shown in the figure, it seems that this organism shares survival and growth characteristics with E. coli, a neutralophile with acid tolerance and resistance mechanisms but not acid-adaptive mechanisms.
From the measurement of survival, which is found at a medium pH only when greater than 4.0, it is obvious that without specialized acid-adaptive mechanisms, H. pylori would not be found in the stomach. It is also clear that any colonization without adaptive mechanisms would be impossible given the pH characteristics of growth. A similar conclusion can be derived from measurements of protein synthesis in vitro, where a correlation is found between protein synthesis and growth, as illustrated.
The organism must have mechanisms preventing a fatal decrease of the proton motive force in acid to survive and even mechanisms for enabling elevation of periplasmic pH in acid to grow in the stomach. Some of these have been identified by both in vitro and in vivo experiments.
Knowledge of the potential difference and pH gradient across the cytoplasmic membrane of H. pylori as a function of medium pH would also enable conclusions as to the nature of the membrane homeostatic machinery necessary for gastric habitation by this organism. The organism is too small for microelectrode measurements of pH or potential difference, but less direct methods using dye probes of these parameters have proved successful.
For example, a dye, bis-carboxyethylcarboxyfluorescein acetomethoxy ester, is loaded into the microorganisms and becomes fluorescent. When this dye is used to measure internal pH at a medium pH of 7.0 using what is called a null point method (i.e., comparing change of fluorescence with all other gradients equal when intracellular pH is made equal to medium pH with the addition of an equilibrating ionophore), internal pH is found to be 8.4 at a medium pH of 7.0. This is equal to a potential difference of approximately -90 mV. A similar measurement of internal pH in E. coli gives an internal pH of approximately 7.8, somewhat lower than that found in H. pylori.
Fluorescent dyes have also been used to measure transmembrane potential. A lipophilic fluorescent dye, the cation di-S-C3-(5), is taken up, and its fluorescence quenches as a function of an internal negative potential. The membrane potential is also calibrated by a null point method, in which the K+-selective ionophore, valinomycin, is added to set the membrane potential to that generated by the transmembrane K+ gradient. Then K+ is added until there is no fluorescence quench (i.e., PD = 0), and then the internal K+ is known, because when there is no membrane potential, [K+]in = [K+]out. From this known value of [K+]in, the membrane potential with the addition of valinomycin and then before addition of valinomycin can be calculated.
At pH 7.0, it is -131 mV (a value also found by studies of distribution of a lipophilic cation). The proton motive force across the inner membrane of this gastric denizen is therefore -221 mV, the sum of the pH gradient and the membrane-potential difference.
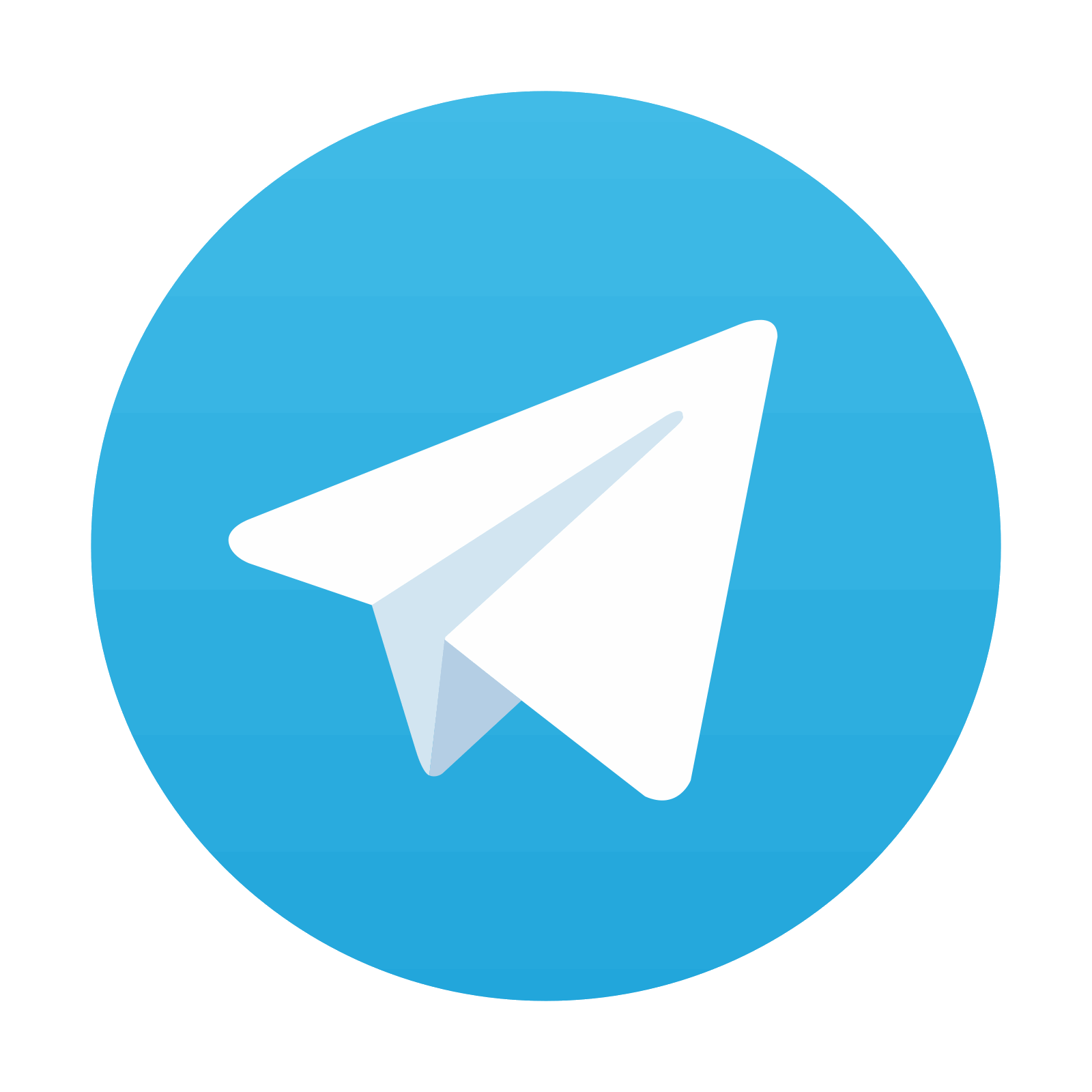
Stay updated, free articles. Join our Telegram channel
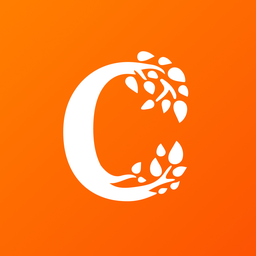
Full access? Get Clinical Tree
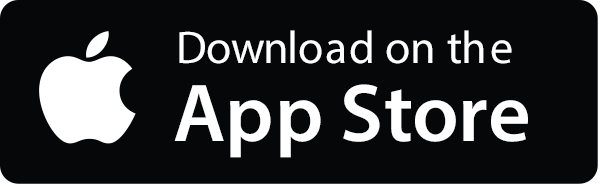
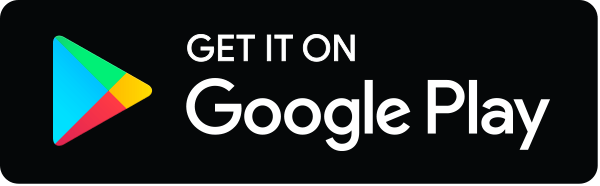