A Brief History and Future Directions for Small Molecule Antivirals for COVID-19 and the Next Pandemic
Mark Namchuk
INTRODUCTION
The scientific response to the coronavirus disease 2019 (COVID-19) pandemic was unprecedented in both its scope and speed. From a therapeutics perspective, this response unfolded in three phases. The first phase was a broad effort to repurpose existing therapeutics and deploy them as potential treatments for COVID-19. The second phase was dominated by the rapid development of antibody therapeutics targeting the spike protein of severe acute respiratory syndrome coronavirus 2 (SARS-CoV-2; see Chapter 8). Finally, novel small molecule therapies, designed for treatment of COVID-19, have begun to emerge. Although not repurposed, many of these molecules owe their origins to previous drug discovery efforts, targeting other viruses.
This chapter focuses mainly on the discovery and development of small molecule antiviral therapies for the treatment of COVID-19. It reviews the outcome of both repurposing and de novo discovery efforts and how these efforts might inform future therapies for the treatment of COVID-19 as well as preparation for the next pandemic. To ensure some level of biological validation, pathways or molecules based solely on computational studies, without at the minimum in vitro data to support the approach, have not been included.
At the outset, it is important to acknowledge that the discovery and development of anti-infectives represents one of the most challenging areas of drug discovery. Effective molecules need to maintain compound concentrations that completely suppress replication of the pathogen throughout the entire dosing period to achieve efficacy and avoid selecting for resistance. For antivirals, this typically requires maintaining drug levels such that the nadir of exposure remains above the in vitro concentration that reduces replication by 90% (EC90), adjusted for free fraction (the amount of drug that remains unbound to plasma proteins or other biologic matrices when administered in vivo). This benchmark has been employed in developing therapeutics for human immunodeficiency virus (HIV), hepatitis C virus (HCV), and SARS-CoV-2.1, 2, 3 and 4 From a therapeutics design perspective, this normally requires compounds of very high potency or that the drug be administered at a high dose in comparison to other therapeutic classes, or both.
DRUG REPURPOSING AND HOST-TARGETED THERAPEUTICS
Repurposing of approved medications was the first approach employed to search for treatments for COVID-19. If successful, this approach can be extremely valuable, given these medicines are often broadly available, cost-effective, and have well-established safety profiles. Within a month of the publication of the identity of the novel coronavirus, SARS-CoV-2, frenetic efforts to develop screens and find compounds with activity against the virus were underway. In retrospect, the result of these efforts is best examined by separating attempts to repurpose molecules that operate through inhibition of a viral target versus molecules that likely exert their effect through hitting a host target. Despite enormous effort, no previously approved molecule is currently approved or authorized as an antiviral for the treatment of COVID-19. However, these studies undoubtedly sped up discovery of the first, effective, direct-acting antivirals.5,6 Host-targeted approaches are reviewed in the next section, whereas the impact of repurposing on accelerating the progression of compounds targeting viral proteins will be covered in the “Direct-Acting Antivirals—Compounds Targeting Viral Proteins” section.
HOST-TARGETED ANTIVIRALS
Preliminary in vitro screens were largely conducted in Vero E6 cells (a monkey kidney cell line), which was a competent host for robust viral replication. A small-scale preliminary report screened seven molecules that had been dosed in humans and had known antiviral properties.7 The study suggested that chloroquine, remdesivir, and favipiravir might be active against SARS-CoV-2 at clinically accessible concentrations of the drug.5,7 Other reports of in vitro activity began to emerge including for ivermectin8 and lopinavir/ritonavir.9
Screens of increasing scale followed, examining broad collections of pharmacologically active compounds. The largest screens conducted employed the 12,000-compound ReFRAME library,10 the 6,700-compound Broad repurposing library,11 and another 3,000-compound library.12 Importantly, hits emerging from these screens began to be counterscreened for their ability to inhibit
viral replication in in cell types besides VERO E6 and significant variability across cell types was observed.12 Careful examination of these data did highlight some common host pathways involved in SARS-CoV-2 replication, but for many active compounds critical liabilities emerged upon more thorough characterization.
viral replication in in cell types besides VERO E6 and significant variability across cell types was observed.12 Careful examination of these data did highlight some common host pathways involved in SARS-CoV-2 replication, but for many active compounds critical liabilities emerged upon more thorough characterization.
Partial Inhibition and Target Redundancy
As noted in the introduction, the ability to achieve near-complete suppression of viral replication is likely an important prerequisite for efficacy. One common observation with compounds in repurposing screens was that inhibition of viral replication was dose responsive, but did not achieve complete inhibition of replication. This suggests that the compound or more broadly the mechanism through which the compound is working to suppress viral replication is redundant. For example, transmembrane trypsin-like serine protease 2 (TMPRSS-2) is a surface-associated protease that plays a role in cleaving the viral spike protein to allow subsequent membrane fusion and viral entry.13 Camostat mesylate is approved for the treatment of pancreatitis in Japan and Korea14 was identified as a TMPRSS-2 inhibitor and had shown activity in repurposing screens previously conducted against SARS-CoV-1 and Middle East respiratory syndrome (MERS).15,16 Characterization of its activity against SARS-CoV-2 showed incomplete inhibition depending on the cell type employed, an observation consistent with later studies showing spike cleavage and subsequent viral fusion could be mediated by calpain and cathepsins.13,17 Similar observations are reported with dihydrofolate reductase inhibitors such as methotrexate, which displayed potent but incomplete inhibition of SARS-CoV-2 replication depending on assay and cell type.18
Interassay Variability
A second finding was variability in potency and level of efficacy based on the cell system used to assess antiviral potency. This finding is more common where the host cell plays a direct role in compound efficacy. For example, nucleoside analogues that inhibit the viral polymerase require the host cell both to remove prodrug groups designed to aid cell permeability and bioavailability and to convert the compound to the active nucleoside analogue triphosphate (see “Nucleoside Analogues” section). This requirement can lead to large differences in the observed antiviral potency depending on the cell type employed.5 Similar variability for host-targeted antivirals has been observed.6 This could guide toward the need to employ more sophisticated cell-based systems (the best mimic of the target organ system, for example), but may also suggest bona fide variability in the effectiveness of the mechanism across different cell types or organ systems. Such biologic variability is commonly observed across human cell lines in other diseases and for example is used to guide indication selection in oncology leading to projects like the DepMap initiative.19 As will be discussed in subsequent sections of the chapter, antivirals that target viral proteins appear to function more consistently across cell types, allowing the in vitro data to be a better predictor of in vivo efficacy.
Drug Exposure Versus Antiviral Potency
To rapidly repurpose a molecule as an antiviral for clinical use, it needs to show antiviral efficacy at or below the dose and exposure attained when the molecule was previously approved for a different indication. Maintaining a concentration of free drug exceeding the EC90 for in vitro replication within this constraint has proven challenging, in particular for drugs with shorter systemic half-lives. Some of the most prominently discussed repurposed molecules for COVID-19 that progressed to clinical development fail on this criterion. For example, hydroxychloroquine was shown to be only partially effective across in vitro systems when dosed at its clinical Cmax.20,21 Ivermectin demonstrated in vitro activity against SARS-CoV-2 with an EC50 in the micromolar range, whereas clinical exposure of the molecule is at concentrations 250-fold below this value.8,22, 23 and 24 Imatinib showed variable antiviral activity based on cell type and was unlikely to achieve adequate exposure versus the viral EC90.25,26
Numerous potential repurposed agents advanced to clinical development in studies of widely varying rigor. Over 200 studies were conducted examining the efficacy of chloroquine and hydroxychloroquine, with the vast majority showing no benefit.6 Ivermectin’s COVID-19 clinical trial history was even more controversial, with one study that showed positive clinical benefit being withdrawn after investigation for academic fraud,27 whereas large randomized controlled trials showed no clinical benefit of ivermectin in symptomatic individuals with at least one risk factor for severe COVID-19.28,29 To date, no compound that had been previously approved has demonstrated significant efficacy as an antiviral for the treatment of COVID-19 when subjected to a well-powered randomized controlled trial.6 As noted earlier, we do not know if the lack of efficacy was a failure of
the mechanism or simply inadequate exposure at the maximum dose previously found to be safe and effective for another indication.
the mechanism or simply inadequate exposure at the maximum dose previously found to be safe and effective for another indication.
Repurposing for Future Pandemics
Examining the outcome of repurposing studies conducted during the COVID-19 outbreak provides several lessons to inform our preparation for the next pandemic. It is important to recognize that the antiviral properties of the molecule being screened may not be derived from the drug’s primary target or could arise from multiple targets the drug engages. This issue is further exacerbated when compounds are tested at super pharmacologic doses, making it difficult to assign the observed activity to the primary target. This mechanistic ambiguity will not impede advancement to the clinic, but does place a high bar on how biologically representative the screens are. Continued development of better and more scalable cell-based assay systems to support rapid profiling of molecules against a novel pathogen would be of value both for initial hit finding and also for screening across assay systems to ensure the antiviral effect is not confined to a single assay system or cell type. From a potency perspective, applying EC90 as the measure of in vitro potency and benchmarking this value to a clinically achievable compound concentration will help ensure the compounds progressed to the clinic have the best chance of success. Early in a pandemic, in vivo models of infection may not be available. However, as they become available, demonstrating an in vivo antiviral effect, at an exposure at or below the compound levels previously achieved in humans, is another important derisking step, in particular for host-targeted agents. Marshaling our clinical resources to run larger trials on fewer, but more promising agents would increase the speed with which agents with bona fide activity can be identified.
DIRECT-ACTING ANTIVIRALS—COMPOUNDS TARGETING VIRAL PROTEINS
SARS-CoV-2 and related coronaviruses encode a large and complex genome. SARS-CoV-2 encodes at least 26 proteins that play essential roles in viral replication or augment the infectivity of the virus through subversion of the host immune response30,31 (see Chapter 2). Repurposing of direct-acting antivirals, initially designed against other viruses, as well as previous research on related coronaviruses unambiguously accelerated the progression toward effective therapeutics against SARS-CoV-2. To date, the two classes of molecules that have demonstrated clinical efficacy are nucleoside analogues and protease inhibitors, which act through inhibition of two essential viral targets, the RNA-dependent RNA polymerase (RdRp) and the 3-chymotrypsin-like (3CLpro) or main protease (Mpro) respectively.
NUCLEOSIDE ANALOGUES
Nucleosides are one of the most broadly deployed classes of antiviral medications including U.S. Food and Drug Administration (FDA)-approved therapies for HIV, HCV, hepatitis B virus (HBV), herpes simplex virus, and others.4,32 Nucleoside analogues are subcategorized based on their detailed mechanism of action and molecular structure. The majority of nucleoside analogues target the viral polymerase directly. They are normally administered in a nonphosphorylated or monophosphorylated form that is activated in cells to the pharmacologically active nucleoside triphosphate (NTP) (Figure 14.1B). Both nucleosides and nucleoside monophosphates are often administrated as prodrugs to improve cellular permeability and oral bioavailability. An important nuance is that nucleoside analogues gain much of their antiviral activity by acting as an enzymatic substrate for the viral polymerase and being incorporated into the growing viral polynucleotide strand.4 This imposes constraints on active molecules to retain structural features of the natural bases, and in practice has also been useful in finding molecules with activity across several viruses.
Post incorporation, nucleoside analogues can function through several mechanisms. Obligate chain terminators lack a 3′ hydroxyl group, hence the polymerase cannot incorporate the next nucleotide in the growing strand. Nonobligate chain terminators retain a 3′ hydroxyl group, yet also do not allow subsequent elongation of the polynucleotide strand. A third category of nucleoside analogues do not cause chain termination, but exert their antiviral effect via other mechanisms, with evidence for error catastrophe (increasing the rate of accumulation of mutations in the viral genome to an extent that the virus becomes nonviable) for certain compounds.4,32
Seven nucleoside analogues of varying mechanisms progressed to late-stage clinical testing for the treatment of COVID-19, all of which were originally designed and/or developed for other uses (Figure 14.1A). These include favipiravir (approved for the treatment of influenza in Japan and Russia), sofosbuvir (approved for the treatment of HCV infection), remdesivir (originally developed for the treatment of Ebola), molnupiravir (originally developed for the treatment of influenza), galidesivir (originally developed for the treatment of HCV infection), AT-527 (originally developed for the treatment of HCV infection), and azvudine (originally developed for the treatment of HIV).
Favipiravir, AT-527, remdesivir, molnupiravir, and azvudine all proceeded to phase 3 trials for the treatment of COVID-19. Remdesivir received FDA approval in 2021 and molnupiravir emergency use authorization in the same year. Azvudine was approved for use in China for the treatment of COVID-19 in 2022. It is unclear if azvudine exerts benefit through a direct antiviral effect, a modulation of the immune response, or some combination thereof.33 Given this ambiguity and lack of available detail for the clinical study data leading to approval,33,34 a more comprehensive assessment will be confined to remdesivir and molnupiravir.
Remdesivir (Veklury)
Remdesivir was one of the first molecules to demonstrate in vitro activity against SARS-CoV-2 and immediately garnered attention as a potential repurposing candidate.7 The nucleoside was originally identified from a screen of about 1,000 nucleosides for activity against RNA viruses including Ebola virus.35,36 Previous studies had shown the parent nucleoside to be weakly active against a number of RNA viruses, including HCV, dengue virus, influenza, and SARS-CoV-1.37 Addition of prodrug moieties to the nucleoside monophosphate improved the compound’s cell permeability and potency37,38 (Figure 14.1A). Upon cellular entry, the prodrug is released in several stages and the nucleoside monophosphate converted to the active NTP, allowing for incorporation into the growing RNA strand by the viral RdRp35,36,39 (Figure 14.1B).
Initial mechanistic studies demonstrated that the NTP is incorporated selectively by viral RdRp as an adenosine analogue and functions through delayed chain termination in filoviruses and coronaviruses.38,40, 41 and 42 For the RdRp from coronaviruses, the NTP is an excellent substrate and is rapidly incorporated into the growing RNA strand with kinetics similar to ATP.41, 42 and 43 The efficiency of incorporation likely allows remdesivir to retain antiviral activity despite the presence of a proofreading exonuclease (ExoN) (see References and NSP 14 section in this chapter). Post incorporation of remdesivir, RNA strand elongation proceeds for three to four additional nucleotides before stalling.42,45 Cryogenic electron microscopy (CryoEM) and biochemical studies suggest this arises from a translocation barrier where the C-1′ nitrile of remdesivir sterically clashes with serine 861 of the RdRp.4,42,45,46 Subsequent detailed kinetic analyses conducted at the single-enzyme level suggest that remdesivir incorporation does not cause chain termination per se, but increases pausing in the synthesis of the new RNA strand, in particular increasing the probability of “backtracking,” where the polymerase reverses direction, disrupting the RNA duplex before proceeding with completing synthesis of the daughter RNA strand.47 Consistent with this hypothesis, full-length remdesivir containing RNA strands can be obtained, in particular at higher NTP concentrations.46 Using this strand as a template decreases the efficiency of incorporation of U when remdesivir serves as a template as well as the rate of incorporation of the subsequent nucleotide.46
Remdesivir has broad activity against RNA viruses. In vitro, remdesivir shows antiviral activity with EC50 values typically below 100 nM against Ebola virus,35,38 Marburg and other filoviruses,38 respiratory syncytial virus (RSV),38 SARS-CoV-1,44,48 MERS,44,48 OC43,49 229E,49 and other coronaviruses48,49 including SARS-CoV-2.7,50,51 It retains in vitro activity across all variants of concern for SARS-CoV-2.52,53 Remdesivir has demonstrated in vivo efficacy in terms of decreased viral burden, clinical signs, and improved histopathologic end points in nonhuman primate (NHP) models of Ebola,38 MERS,54 and SARS-CoV-2 infection.55
Remdesivir has a high barrier to resistance across all viruses tested to date with mutations identified in the RdRp and normally accompanied by a fitness penalty.44,56 In vitro resistance selection conducted against SARS-CoV-2 gave rise to a single point mutation in the viral polymerase, E802D, which increased the in vitro viral replication of EC50 by approximately 2-fold.57 Sequencing of virus post remdesivir treatment in NHP efficacy studies failed to identify resistance mutations.38,54 Clinical resistance to remdesivir in treating COVID-19 has not generally been observed, although a case
study of an immunosuppressed patient receiving remdesivir treatment noted the emergence of virus with the E802D mutation.58
study of an immunosuppressed patient receiving remdesivir treatment noted the emergence of virus with the E802D mutation.58
Drug development efforts for remdesivir initially focused on treatment of Ebola infection.36 Although the Ebola trial ultimately proved unsuccessful, it did establish both a dose and dose regimen for the drug.59 Clinical development of remdesivir for COVID-19 initially focused on hospitalized patients. Conflicting evidence emerged as to the clinical efficacy of remdesivir in this setting, with a large National Institute of Allergy and Infectious Diseases (NIAID)-led study demonstrating efficacy,60 whereas studies conducted by the Discovery and Recovery consortia did not.61,62 Notably, treatment with remdesivir in the outpatient setting was 87% effective in preventing hospitalization of patients with at least one risk factor for severe COVID-19 when administered within 7 days of initial symptom onset.63 This provides an important insight into the connection of disease stage and antiviral efficacy, arguing substantial benefit may be confined to the outpatient setting. Remdesivir’s intravenous (IV) route of administration limits its use in this setting.
Remdesivir is FDA approved for the treatment of COVID-19 requiring hospitalization.64
Molnupiravir (EIDD-2801, MK-4482)
Molnupiravir is an orally available 5′ isopropyl ester prodrug of β-D-N4-hydroxycytidine (EIDD-1931, NHC), a ribonucleoside analogue (Figure 14.1A). Although identifying molnupiravir’s utility in treating COVID-19 falls under the broader umbrella of compound repurposing, it should be noted that the molecule had not been previously tested clinically and hence did not have the advantages of clinically established efficacy or tolerability.
EIDD-1931 (NHC) arose from efforts at Emory University specifically focused on the design of nucleoside analogues with a broader spectrum of action based on sequence conservation among polymerases, initially screening for agents for activity against Venezuelan equine encephalitis virus.65 NHC has demonstrated in vitro antiviral activity across numerous RNA viruses with EC50 values in the micromolar to nanomolar range reported for norovirus,66 chikungunya virus,67 Venezuelan equine encephalitis virus,68 Ebola and Marburg virus,69 influenza,70 and several coronaviruses including NL-63,71 SARS-CoV-1,72,73 MERS,73 and SARS-CoV-2.73 Although EIDD-1931 was orally bioavailable in rodents, concerns about enterocyte stability and pharmacokinetics (PK) in NHPs prompted development of the prodrug, molnupiravir (EIDD-2801)65,70 (Figure 14.1A). In preclinical animal model studies, molnupiravir has demonstrated in vivo antiviral efficacy against influenza,70 SARS-CoV-1, MERS, and SARS-CoV-2 infection.70,74, 75 and 76 Molnupiravir retains activity across all variants of concern for SARS-CoV-2.52,53,77
Molnupiravir is incorporated into the RNA strand via the RdRp but does not cause chain termination. The mechanism of action for molnupiravir is ascribed to error catastrophe causing a selective accumulation of mutations within viral RNAs until the resulting virus is inviable, though other mechanisms may also contribute.4,68,73,78,79 A similar mechanism has been suggested for favipiravir.80,81 Modification of the cytidine to NHC allows for the base to exist in two tautomers, enabling base pairing with both A and G.82 Biochemical studies of the RdRp from SARS-CoV-2 demonstrate NHC triphosphate can be efficiently incorporated during RNA transcription when the template codes for G and to a lesser extent A.79,82, 83 and 84 Template strands containing incorporated NHC can subsequently incorporate either G or A.79,82,83 Studies in a number of viruses, including SARS-CoV-2, show that the NHC base increases the error frequency in viral RNAs with a preference for A to G, G to A, C to U, U to C transitions.68,70,73 Here again, efficient incorporation into viral RNA may allow NHC to avoid excision via proofreading ExoNs.85 NHC has a high barrier to resistance, and changes in drug sensitivity have not been correlated with a signature mutation.68,70,85
Clinical development of molnupiravir for COVID-19 included dose-ranging studies and two phase 2 programs examining molnupiravir efficacy in hospitalized patients (MOVE-in) and in the outpatient setting (MOVE-out).86 Analysis of both studies suggested clinical efficacy was confined to the highest dose tested (800 mg) and only to patients in the outpatient setting.87,88 The MOVE-in study was terminated for futility.87
Clinical studies demonstrated that molnupiravir decreased the rate of hospitalization by 29% in patients with COVID-19 with at least one risk factor for developing severe disease when treatment is initiated within 5 days of symptom onset.89 No evidence of clinical resistance to the compound has been observed to date.90
MAIN PROTEASE INHIBITORS
Coronaviruses are the largest and most complex RNA viruses. Post cellular entry, viral proteins are expressed as two large polyproteins, pp1a and pp1ab, encoding 11 and 16 viral proteins, respectively.31,92 These polyproteins are processed by two virally encoded cysteine proteases, the papain-like protease (PLpro) and the 3-chymotrypsin-like protease or main protease (3CLpro or Mpro).31,92 PLpro mediates processing of the amino-terminal portion of the polyprotein, cutting at three sites to release NSP1-3, whereas Mpro cleaves at 11 sites to release NSP4-16. Both protease functions are essential for viral replication.
Analagous to the nucleoside analogues, a critical driver of the rapid advancement of Mpro-based therapeutics was previous drug discovery efforts targeting viral proteases. In particular, previous studies aimed at discovering treatments for SARS-CoV-1, feline infectious peritonitis, and HCV provided advanced starting points for SARS-CoV-2-targeted Mpro inhibitors. Subsequent design efforts were facilitated by extensive work characterizing the structure of SARS-CoV-2 Mpro93 and the discovery that orally available antiviral protease inhibitors such as boceprevir had activity against the SARS-CoV-2 Mpro.94 As of this writing, at least five Mpro inhibitors had progressed to at least phase 2 clinical development for the treatment of COVID-19. Two molecules, nirmatrelvir and ensitrelvir, have progressed to authorization and phase 3 clinical studies, respectively, and are reviewed in greater detail.
PF-07304814
The first Mpro inhibitor to proceed to clinical development for COVID-19 was PF-07304814, a phosphate prodrug of PF-000835231 (Figure 14.2). The molecule arose from a previous drug discovery program targeting the Mpro from SARS-CoV-1, but was not progressed to clinical development as the outbreak abated.95 PF-000835231 is a peptidomimetic design and exemplifies several molecular features common to most Mpro inhibitors: a “warhead” moiety designed to covalently engage the catalytic cysteine (in this case a hydroxymethyl ketone), and a γ-lactam as a glutamine mimetic at P1 (Figure 14.2). Given the level of substrate conservation across coronaviruses,96, 97 and 98 a level of cross-inhibition between SARS-CoV-1 and SARS-CoV-2 is unsurprising and consistent with the broader spectrum activity seen with other similar molecules, with activity across picornaviruses, noroviruses, and coronaviruses.99, 100 and 101 For example, GC376 designed to target the Mpro from feline coronavirus (Figure 14.2)102,103 is active against SARS-CoV-2 infection, with low micromolar in vitro antiviral potency.104
PF-07304814 was clinically tested against SARS-CoV-2 as part of the ACTIV-3 clinical program.105 PF-07304814 is dosed IV and hence confined to use in the inpatient setting. Development was halted based on the “totality of the data” in February 2022.106
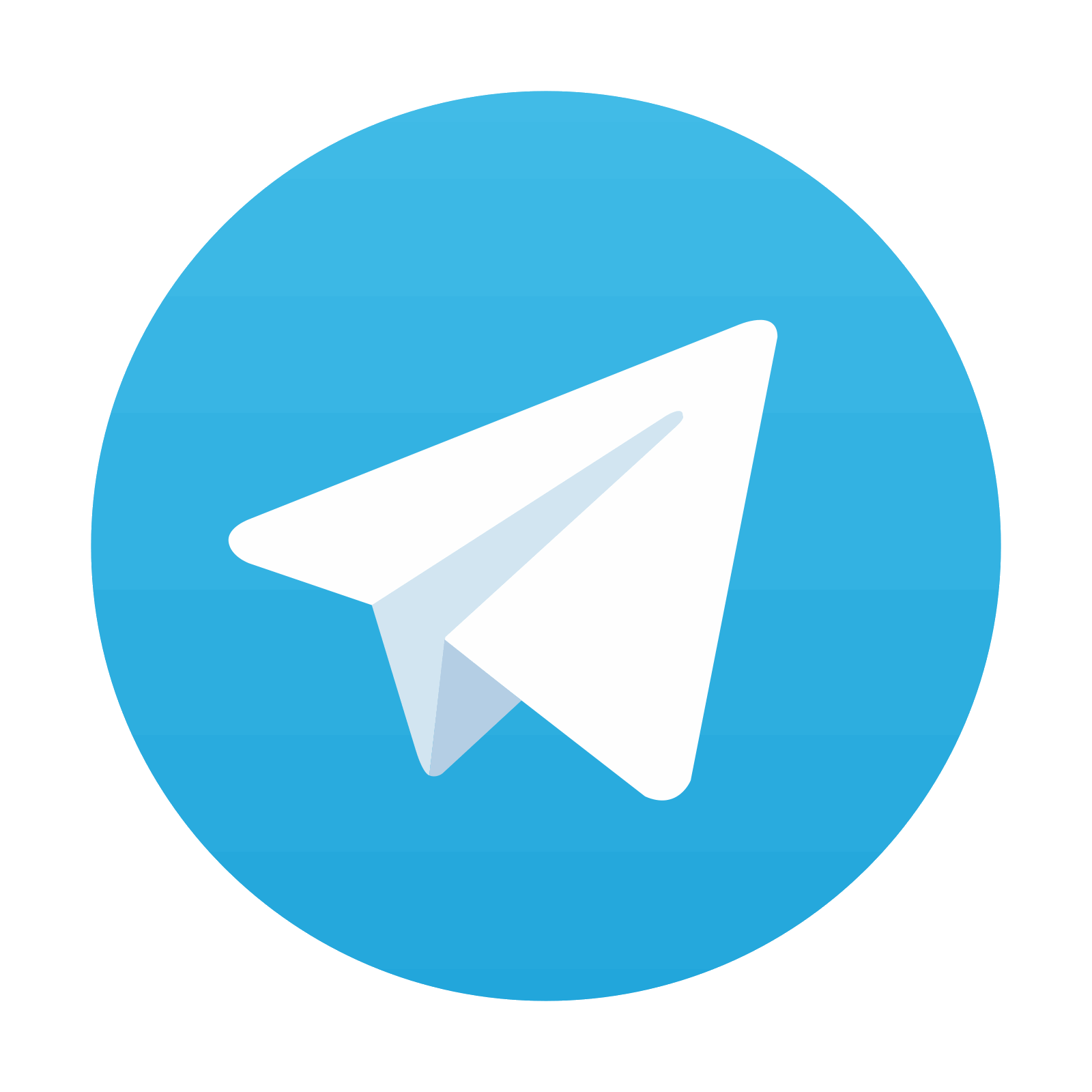
Stay updated, free articles. Join our Telegram channel
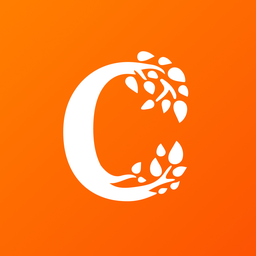
Full access? Get Clinical Tree
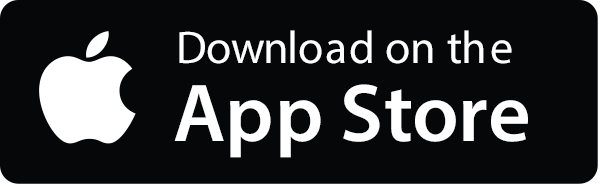
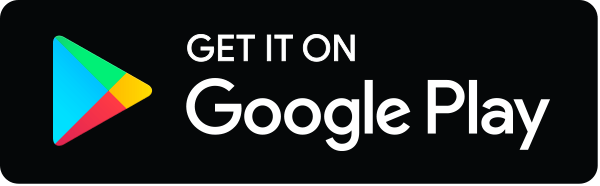